Introduction
Materials science and 3D printing
As discussed in Chapter 1 , materials for 3D printing include polymers, ceramics, metals, and composites. The materials used to fabricate a 3D part are dependent on the performance, or the “requirements” of the final part for its application. Material selection is also dependent on the 3D-printing technology used, as not all materials can be manufactured using all available 3D-printing methods. Thus, the intersection of material science with 3D printing has grown into a vast and popular field since the 2000s. Continued work in this field will produce a better understanding of materials, manufacturing, and their use in medical applications.
Processing-structure-property-performance relationships
In material science, processing-structure-property-performance relationships define the correlation between the way a part is made and the resulting behavior and application of the part. Processing refers to the steps a raw material undergoes during the manufacture of a final part. This can include both primary and secondary processing steps. For a 3D-printed part, additive manufacturing is typically the primary processing step in which a raw material in the form of a filament, resin, powder, or other type, is converted to a 3D part. Secondary processing of the part may include heat treatment, surface treatment, or other additional steps to achieve the desired structure and therefore properties. The performance, or function, of the part is dependent on its various properties. In orthopedic applications, performance is typically driven by a tradeoff between the mechanical and surface properties of a given application. For example, a hemiarthroplasty implant must be high strength and fatigue resistant, as well as have a bearing surface with a low coefficient of friction to minimize wear. These relationships help material scientists and engineers to select materials for a given application, as well as to discover new materials.
Materials selection
As described earlier, processing of a material defines its structure, and thus the resulting material properties. In load-bearing orthopedic applications, mechanical (structural) performance is typically a priority when designing an implant. In additive manufacturing, due to the wide selection of materials and processing technologies, a top-down strategy can be employed to first define the desired properties of a part for a given application. For example, for a tibial tray of a total ankle prosthesis, engineering requirements may prescribe a minimum strength before failure, as well as a coefficient of friction of the surface interfacing with the polyethylene insert, amongst other requirements. These properties and their interrelationships must be considered in order to define the appropriate processing steps to achieve the end goal.
Most importantly, these properties help to define the materials that can be used for the part. Material selection charts, also often called Ashby plots, are often used in these cases to identify materials that can meet defined the specifications for a given application ( Fig. 3.1 ). The following sections give an overview of the current state of the art for materials produced by 3D printing for medical applications and biomedical research.

Aside from selecting the type of material (metals, polymers, ceramics, or composites thereof), there are other important material and final implant characteristics that need to be addressed for the successful fabrication of a 3D-printed orthopedic device ( Fig. 3.2 ).

Metals
From the perspective of orthopedic implant applications, the first 3D-printed implants were metallic. Given the historical use of titanium and its alloys for skeletal reconstruction, the development of these materials was at the forefront of the adoption of additive manufacturing technologies in the orthopedics industry. The adoption of 3D printing has been dominated by titanium alloy, with 40% of such medical devices cleared by the United States Food and Drug Administration (FDA) between 2010 and 2015. Implants fabricated from commercially pure (unalloyed) titanium and cobalt chromium (CoCrMo) alloy have also been developed and cleared, albeit to a far smaller degree.
Titanium and titanium alloys
Titanium, both commercially pure (CP) and the most common alloy, Ti6Al4V, have long been used in the human body for various load-bearing and nonloaded applications. In fact, the first 3D-printed implant cleared for use by the FDA was a titanium alloy acetabular hip cup manufactured by Exactech, Inc. Due to its high strength and fatigue resistance, Ti6Al4V is the standard choice for load-bearing orthopedic implants. The manufacture of porous implants with complex, interconnected lattices has been a primary use case for additive manufacturing in implants. Of the devices produced by additive manufacturing (AM) with a porous lattice, 72% are Ti6Al4V. These include devices such as spinal fusion cages, osteotomy wedges, and arthroplasty components, where osseointegration of the implant is critical to a successful clinical outcome. Thus, research efforts at the intersection of AM and the biomedical field are focused on optimization of porous lattices to improve the efficacy of such implants. This includes investigation of the tradeoff between design and manufacturing, the mechanical properties of porous scaffolds, and the associated in vivo performance. ,
Nickel titanium (NiTi) is another alloy used in medical device applications. To date, no additively manufactured implants fabricated from NiTi have been cleared by the FDA. However, there many research efforts have been dedicated to overcoming the challenges related to additive manufacturing of the alloy. AM has also led to the development of new alloys that are designed with compositions that are well suited for the additive process. One such example is titanium niobium (TiNb) alloys, which have received increasing research attention due to their ability to reduce the bulk material modulus while maintaining high strength similar to Ti6Al4V. , Challenges in the 3D printing of titanium and its alloys include the sensitivity to oxidation, which is discussed further here. This is a particular challenge for NiTi, where even small changes in atomic composition drastically effect the material’s properties, and the ability to achieve super elasticity.
Cobalt alloys
Cobalt chromium (Co 28 Cr 6 Mo) is a high-strength alloy with a low coefficient of friction, which facilitates its use in articulating applications where reduction of wear is critical ( Fig. 3.3 ). While its adoption into the additive manufacture of implants has lagged behind titanium alloys, there are an increasing number of implants that use this alloy, including the talar implant for a total ankle arthroplasty system (Wright Medical Infinity TAR). Similar to titanium, CoCrMo alloy forms a spontaneous oxide layer. Thus, similar solutions to allow high-temperature processing with oxidation of the surface are employed. Additionally, the resulting surface of as-printed parts requires significant postprocessing to reduce the roughness to within the specifications defined for articulating arthroplasty components (<0.05 μm per ASTM 2033).

Other metals
Stainless steels, including 17-4 and 316L, are commonly used in surgical applications for both implants and instruments. The use of powder bed fusion (PBF) for producing stainless steel implants has been less widely adopted than for titanium alloys. However, opportunity exists to use 3D printing for the production of stainless steel plates or other orthopedic applications.
3D printing of precious metals has also been demonstrated in the scientific literature; for example, pure silver bone scaffolds which exhibit antibacterial properties. Other work has investigated the addition of copper (Cu) or gold (Au) to traditional alloy systems to impart antimicrobial properties to the implant. These additions to the alloy system also have an effect on the mechanical properties of the materials, which needs ongoing research to be better understood.
A number of bulk metallic glass systems have also been under investigation for the AM of implants, including Ti 47 Cu 38 Zr 7.5 Fe 2.5 Sn 2 Si 1 Ag 2 and Zr 52.5 Cu 17.9 Ni 14.6 Al 10 Ti 5 . The advantage of bulk metallic glass is its ability to reduce stiffness while maintaining a high strength. Additional degradable metals such as magnesium, zinc, and iron-based materials have also been discussed for their use in bone-regeneration applications in the “Degradable Materials” section.
3D-printing technologies for metals
Powder bed fusion
PBF has been the primary technology adopted for 3D printing of medical devices. PBF of metallic materials can be further divided into subcategories of technologies based on the high energy source used to achieve fusion of the powder material. The two most common are electron beam melting and laser PBF. While both technologies have been used to produce metallic medical implants and there are advantages and disadvantages to each depending on the end part and its application, laser PBF has seen wider adoption. This wider adoption can be attributed to its ability to achieve smaller feature sizes and control complex geometry, which are advantageous for the fabrication of complex porous lattices to allow for osseointegration.
While PBF has unlocked an unprecedented opportunity to produce medical devices, there are some processing-related challenges that must be considered. First, the sensitivity of reactive metals to oxidation at high temperatures must be managed. In PBF, this is overcome by use of inert gases and/or a vacuum in the build chamber to reduce the risk of oxidation. Typically, oxygen levels are well below 100 ppm throughout the duration of the build. Further, the metallic material produced from PBF usually has a microstructure that differs from that of the wrought metal. Due to the layer-wise process, anisotropy in the grain structure is often observed, as well as a non-equilibrium phase. For example, titanium alloy is a biphasic material and the as-printed microstructure is a non-equilibrium alpha resulting from rapid heating and cooling during the PBF process. To homogenize the material microstructure, reduce residual thermal stresses, and improve the material properties, PBF parts typically require postprocessing thermal treatments. These include standard stress relieving, high-temperature annealing, as well as hot isostatic pressing (HIP) processes. Lastly, the as-printed surface of parts resulting from PBF can have roughness (R a ) ranging from 5 to 50 μm due to the adherence of partially fused powder particles. While in some osseointegration applications this topography may be favorable, in those at risk of high wear, the surface of implants must be treated through chemical or physical methods.
Others
While PBF technologies have dominated the AM of implants to date, other technologies are poised to offer advantages for the production of metallic implants. Directed energy deposition (DED) has been investigated for printing of multimaterial components due to its ability to switch between feedstock more easily, which allows the local deposit of different materials or the introduction of material gradients. , One such example would be a hip stem with a porous titanium alloy stem and a CoCrMo head for articulation.
Similarly, material extrusion and binder jetting (BJ) of metallic parts have been demonstrated in both research and commercial settings. However, the material selection and geometric complexity are currently limited in these processes. Further, both technologies require a postprinting workflow that includes debinding and sintering steps. One major hurdle for these technologies in implantable medical devices will be complete removal of the binder material to ensure no contamination occurs in the body.
Polymers
Introduction
A polymer is a macromolecule composed of smaller repeating units known as monomers. A polymer is formed when monomers are linked together by a primary bond that is typically covalent in nature. Colloquially, a subset of synthetic polymers is better known as plastics. Polymers are of significant interest in medical technology owing to their unique characteristics and large range of properties. For example, the mechanical properties of polymers can range from being akin to gel, rubber, or hard plastic. Many common polymers also possess good processability, making them amenable to 3D printing. These qualities have led to a vast number of commercial products and scientific research that utilize 3D-printed polymers for medical applications. In orthopedics, commercial applications of 3D-printed polymers have ranged from simple surgical guides and instruments to a bioresorbable bone graft cage implant. The types of 3D-printed polymers available for orthopedic implants are limited due to stringent biocompatibility requirements but a much larger range of polymers is available for nonimplant applications (e.g., surgical guides, surgery planning aids, orthoses).
The properties of a polymer are largely determined by its chemistry, structure, and morphology. By varying these aspects, polymers can be tuned to achieve specific objectives and improve the performance of a device. The chemistry of a polymer is the combination of atoms contained in a polymer chain and the manner in which they are bonded to one another. The structure or configuration of a polymer refers to the way the atoms in a polymer are arranged, which could vary in a polymer with the same chemistry, such as in linear versus branched polymers. The morphology of a polymer is the arrangement of polymer chains in relation to all other polymer chains and any ordering associated with it, and the morphology is strongly related to its processing history. In addition to these three factors, the property of a polymer can also be altered by using additives to achieve specific goals. For example, plasticizers are commonly added as an additive to promote plasticity and reduce the brittleness of a polymer.
Nondegradable polymers used in orthopedics 3D printing
From the perspective of orthopedic applications, there are some 3D-printable polymers that are frequently in use in both scientific research and commercial products. These polymers are described and their applications in the context of orthopedics are presented in the following section. All the polymers shown here are discussed in the context of their use as a nondegradable material, which is defined as a material that does not degrade appreciably within its service life.
Most polymers commonly used for 3D printing are not suitable for implant applications. The requirements for implant materials are much more stringent than nonimplants and include specifications spanning from biocompatibility and physical properties, to mechanical properties. Generally, nonimplant applications (e.g., surgical planning aids) only require two properties: safety for short-term contact and suitable mechanical properties ( Fig. 3.4 ).

In this chapter, synthetic polymers are categorized into thermoplastics, thermosets, and photopolymers. This classification reflects the nature of their processing, which in turn strongly determines the 3D-printing methods available to them.
Thermoplastics
Thermoplastics are polymers that can be melted when exposed to temperatures above their melting temperature. In thermoplastics, the polymer chains are held together by secondary bonds which form physical crosslinks such as hydrogen bonds, which are reversible. The physical crosslinks dissipate when heated past a critical temperature but reform when cooled, granting thermoplastics their reprocessable nature. Reprocessable refers to the capability of an already shaped polymer to be melted and reshaped. Because of these properties, thermoplastics lends themselves well to fused filament fabrication (FFF) and PBF 3D printing.
Nylon
Nylon is a colloquial name for a group of polymers based on aliphatic polyamides. An aliphatic polyamide is a polymer with aliphatic repeating units connected by amide bonds. There is a large variety of nylon available, the most common being PA6, PA66, PA11, and PA12. Nylon is a semicrystalline thermoplastic polymer with high toughness and strength, and is steam sterilizable. It also has a wide processing window, allowing it to be processed in a large variety of ways. Owing to this processing window, current PBF printing of polymers mainly uses nylon. In medical devices, nylon is often used in sutures and in short-term devices such as balloon catheters; nylon is rarely used long term. In orthopedics, 3D-printed nylon sees use in nonimplant applications such as surgical guides and tools due to its excellent mechanical properties and its amenability to PBF printing.
Poly(aryl ether ketone) polymers
Poly(aryl ether ketone) (PAEK) is a relatively new family of thermoplastic that is composed of a backbone of paraphenylene groups interspersed with ketone and ether groups. Prominent polymers in the PAEK family include poly(ether ether ketone) (PEEK), poly(ether ketone) (PEK), and poly(ether ketone ketone) (PEKK). Of the members of the PAEK family, PEEK is the most commonly used in commercial applications. PEEK is a polymer with a high melting temperature (allowing for steam sterilization), radiolucency, and excellent mechanical and chemical resistance properties. PEEK also has a stiffness that is closer to bone than metal, minimizing stress shielding. In medical devices, PEEK has vast applications, particularly in orthopedics. It is also one of the few polymers commonly used for load-bearing orthopedic implants. PEEK has a long and established history of clinical use, exhibiting osteoconduction and biocompatibility. Some examples of PEEK implants include internal fixations for bones, spinal cages, and craniofacial implants. PEEK also has nonimplant applications as surgical instruments and its stability allows for autoclaving, enabling sterilization, and repeated use.
The most common processing method for PEEK is injection molding, which is commonly used as a benchmark for 3D-printed samples. PEEK is amenable to both extrusion and PBF 3D printing. 3D printing of PEEK is a frequent research subject because of its perceived potential for commercial applications. In past studies of PEEK FFF 3D printing, a wide variety of resulting properties have arisen because of the wide-ranging testing parameters and set up used by the authors. In general, printed PEEK is highly anisotropic and has vastly inferior mechanical properties to injection-molded PEEK. , Many approaches have been proposed to mitigate the reduction in mechanical properties, such as annealing/heat treatment, adjustments to the printing environment, and other print parameter optimizations ; however, none have yielded significant improvement on the observed anisotropy. Currently, no commercial PEEK implant is 3D printed via FFF. Studies on PBF 3D printing of PEEK have generally demonstrated mechanical properties that are superior to FFF printing. , However, although these studies did not test for anisotropy, printed PEEK is expected to be anisotropic, as has been shown in other PBF-printed materials. , Currently, to the best of the authors’ knowledge, there is no commercial PBF-printed PEEK implant.
PEKK is another member of the PAEK family that is useful in orthopedic implants. Chemically it is similar to PEEK, but with one ether group and two ketone groups interspersing the paraphenylene group backbone. In orthopedics, PEKK is used in similar applications to PEEK because its properties are in many ways comparable. One of the earliest 3D-printed polymer implants cleared by the FDA is a craniofacial device (OsteoFab, Oxford Performance Materials) fabricated out of PEKK via selective laser sintering (SLS). Currently, there are also a number of FDA-cleared PEKK 3D-printed load-bearing spinal implants (SpineFab, Oxford Performance Materials; Tetrafuse, RTI Surgical) fabricated via SLS ( Fig. 3.5 ). A key advantage for PEKK 3D printing compared to PEEK 3D printing is printed PEKK’s superior properties, a direct result of its thermal behavior which is suited for PBF printing.

Other thermoplastic polymers
Polymers that find some use in 3D printing for orthopedic research and commercial devices are discussed here:
- 1.
Acrylonitrile butadiene styrene (ABS): ABS is one of the most common polymers for FFF 3D printing, especially in a home 3D printer setting. Outside of medical applications it is often used in products such as toys and enclosures for consumer electronics. In medical devices, ABS finds the most use outside of implants. In orthopedics, FFF 3D-printed ABS is used in the fabrication of surgical planning aids as it is sterilizable using ethylene oxide.
- 2.
Polylactic acid (PLA): PLA is another common polymer in FFF 3D printing along with ABS. It is an aliphatic polyester composed of lactic acid. It has favorable properties for processing via extrusion and molding. In medical devices, PLA is most often used in implant applications requiring bioabsorbability. In orthopedic applications without need for bioabsorbability, FFF 3D-printed PLA has been used in surgical planning aids.
- 3.
Ultra-high-molecular-weight polyethylene (UHMWPE): UHMWPE is a common engineering polymer with excellent mechanical properties and good wear resistance, self-lubricating properties, and biocompatibility. In orthopedics, it is commonly used in hip, knee, and spine implants for articulating surfaces. The 3D-printing method of choice for UHMWPE is PBF. However, its high melt viscosity hinders optimal printing via PBF, resulting in inferior properties. At its current state, 3D printing of UHMWPE appears to be unsuitable for use in common UHMWPE orthopedic implants.
- 4.
Polymethyl methacrylate (PMMA): PMMA, also known as bone cement, is a ubiquitous polymer in orthopedics. PMMA is also used in other implant applications, such as in craniofacial implants (e.g., PMMA Customized Implant, Stryker). Its thermoplastic nature allows it to be printed using extrusion and PBF methods. PMMA can also be printed via BJ. However, the printed parts possess significantly inferior mechanical properties and/or lower density compared to injection-molded samples.
- 5.
Polypropylene (PP): PP is a semicrystalline polymer composed of propylene monomers and the second most produced thermoplastic in the world behind polyethylene. PP is a widely used material in FFF 3D printing. It is also one of the most used polymers in medical devices, frequently seen in sutures, packaging, noncontact devices, and implants. In orthopedics, an example of PP application is in ligament augmentation devices. SLS-printed PP shows properties close to injection-molded PP.
- 6.
Thermoplastic polyurethane (PU) : PU belongs to a large family of polymers that are used in a large variety of industries. PU’s main characteristic is its urethane links that joins its monomers together. Polycarbonate urethane (PCU) is a member of the PU family that has garnered much attention for its potential use in orthopedics, particularly as articulating surfaces for knee implants, , and has been shown to be very amenable to FFF 3D printing, having similar properties to injection-molded samples.
- 7.
Polysulfones (PSUs): PSUs are a group of high-performance polymeric materials. Similar to PAEKs, PSUs possess high hydrolytic resistance, high temperature resistance, and good mechanical properties. They are often used in reusable devices requiring sterilization such as surgical trays and sizers for implants. Commercial PSU 3D-printing filaments are available for extrusion printing, such as Ultem 9085 (Stratasys). Extrusion-printed PSUs have shown potentially similar properties to injection-molded PSUs in past studies.
Thermosets
Thermosets are chemically crosslinked polymers with a highly restricted chain motion. Because of the covalent nature of the crosslinks, thermosets are not reprocessable, unlike thermoplastics and their physical crosslinks.
Silicone
Silicone, or polysiloxanes, are polymers containing a siloxane (Si-O-Si) linkage. Silicones are used in many applications owing to their very versatile properties. Medical device applications for silicones are particularly vast because of their biocompatibility and chemical stability. An example of clinical applications of silicones include contact lenses and catheters. In orthopedics, polysiloxanes are used in hand and foot joint implants. 3D printing of polysiloxanes is commonly performed via direct extrusion and a base silicone material is typically mixed with substances to achieve a consistency that is suitable to be used as a printable ink.
Photopolymers
Photopolymers are polymers that are crosslinked under exposure to light of a particular wavelength and are printed using vat photopolymerization and material jetting (MJ). Most photopolymers are not reprocessable, hence they are often a subset of thermoset polymers. A photopolymerization system is typically composed of three components: a mixture of monomers and oligomers, photoinitiators, and other additives such as stabilizers and photosensitizers. Most commercial mixtures are proprietary, and their list of components are not readily searchable. The most common photopolymer systems are described here. It is important to note that, in practice, these different systems are often combined into a complex mixture to improve properties.
Currently, commercial photopolymers find most of their applications in nonimplant devices such as surgical planning aids and guides. Commercial photopolymers have a wide range of mechanical properties and are suitable for most nonimplant applications. However, to the best of the author’s knowledge and at the time of writing, no commercial photopolymer has been present in an FDA-cleared human implant.
Acrylate and methacrylate-based systems
(Meth)acrylate-based photopolymerization is the earliest type of system developed for vat photopolymerization (VP) and remains the most common. (Meth)acrylate-based systems polymerize via free radical photopolymerization (FRP), which is a polymerization approach where a polymer is formed by the addition of monomers into a propagating radical chain. An example resin mixture is a combination of urethane acrylate, urethane methacrylate, trimethylolpropane triacrylate, and other reactive diluents along with photoinitiators for use in continuous liquid interface production (CLIP) 3D printing. (Meth)acrylate-based resins are very versatile and are used in many 3D-printing applications such as for shape-memory polymers, tough polymers, natural polymers, and functional polymers. (Meth)acrylate resins have a high rate of polymerization and exhibit long-term stability, but generally result in parts that are brittle. They exhibit significant shrinkage when cured, and as a consequence they can also exhibit deformations such as curling. Some pure (meth)acrylate resins also show significant gelling even at low conversions, inducing high viscosity and limited flow of resin for optimal printing. For these reasons, meth(acrylate) resins are often combined with epoxy-based resins to improve properties.
Epoxy-based and vinyl ether-based systems
Epoxy and vinyl ether-based systems are both facilitated via cationic photopolymerization. Cationic polymerization is chain-growth polymerization via carbocationic growing species. Examples of common epoxy monomers include 3,4-epoxycyclohexane methyl 3,4-epoxycyclohexylcarboxylate (EPOX), bisphenol A diglycidyl ether (DGEBA), and 1,4-cyclohexane dimethanol divinyl ether (CDVE). Epoxy-based systems and other similar ring-opening polymerization systems tend to show minimal shrinkage compared to acrylates and they are often mixed with acrylates to allow for thermal post curing and to minimize shrinkage. Epoxy resins also show less inhibition by oxygen, allowing for a lower photoinitiator concentration. However, epoxy resin photopolymerization reactions tend to proceed more slowly compared to acrylate’s free radical polymerization.
Thiol-ene and thiol-yne systems
Thiol-ene and thiol-yne systems are based on the reaction between thiols and carbon-carbon double bonds and triple bonds, respectively. These systems have a couple of advantages over (meth)acrylate systems in that they display less shrinkage and less oxygen inhibition. They also demonstrate improved biocompatibility, hence the significant interest in using them for applications requiring biocompatibility and degradability. , However, these systems tend to have a poor shelf life and bad odor.
3D-printing processes for polymers
3D printing of polymers is available in a large variety of approaches because of the large range of available polymer properties. In this section, the most common methods of polymer 3D printing are described. Important considerations for each method are presented in the context of printing parameters, mainly from a materials perspective.
Extrusion
The main advantages of extrusion-based polymer printing are its simplicity and a large library of material that is available for use, some of which possess an established clinical use history. However, structures with overhanging sections are difficult or impossible to print via extrusion and should be minimized during device design.
Printing parameters that are important to polymer extrusion are temperatures, and print pathing and orientation. Typically, the overarching objective of adjusting these parameters are to (1) achieve good mechanical properties, and (2) print parts with good fidelity. In achieving good mechanical properties, layer bonding is the primary consideration. For good part fidelity, the extruded polymer needs to maintain its shape in a predictable way when extruded out to form layers.
Nozzle and bed temperature are considered one of the most important print parameters for melt-based extrusion. Excessively high temperature leads to polymer degradation and undesired spreading of the molten polymer, while inadequate temperature leads to low polymer flow and weak layer bonding. In addition, temperature also has a more nuanced effect on printed polymers, such as by affecting crystallinity. Print pathing and orientation are also major factors in improving print quality. Print pathing includes aspects such as layer thickness, path width, raster pattern, and many others, whereas print orientation refers to the orientation at which the object is printed. Broadly speaking, parameters that maximize part density (such as smaller layer thickness) and direct mechanical loads away from layer bonding surfaces (such as via raster pattern adjustment) will improve quality.
There are a vast number of parameters that can be adjusted for extrusion printing. All these parameters are interrelated; therefore, it is important to evaluate each printed part using the exact same parameter as production parts, including the same environment, same device, and same material. In orthopedics, extrusion printing has found the most use in surgical planning models. The simplicity of extrusion systems also means it can be used on site in clinical settings. At the time of writing, to the best of the author’s knowledge, no commercial orthopedic device is fabricated via extrusion printing.
Vat photopolymerization
VP printing is a process unique to polymers. The main advantage of VP is its ability to print complex structures. VP-printed parts are also accurate, with a smooth surface finish. This has led VP parts to find use in applications that require accurate form and fitting. However, photopolymers tend to be nonbiocompatible for implant use. Scarce clinical usage history is a major obstacle for use as an implant by device developers. In comparison to injection-molded parts, VP parts have lower durability and strength, in addition to the anisotropy common with 3D-printing techniques.
From a processing perspective, an ideal resin mixture for VP would have low viscosity, minimize undesired light leakage, polymerize rapidly, and have no shrinkage when cured. The formulation of the resin strongly affects this. Important parameters in VP include cure depth and scan pathing. Cure depth is a commonly used VP measure that includes the effect of laser power, laser penetration, laser speed, resin cure-threshold, and laser spot size. Along with cure depth, scan pathing is an important parameter that is able to minimize residual stress and shrinkage when adjusted well. It is also worth noting that VP systems can use different types of radiations (e.g., ultraviolet [UV], visible light, or infrared) to initiate photopolymerization.
Research directions in VP include novel VP approaches such as two-photon microprinting. In the context of medical devices and orthopedics specifically, there is much interest in the development of novel photopolymers that are biocompatible for implant application. Some past research on this subject includes the development of biocompatible hydrogels, , biodegradable scaffolds, filler-added resins, and so on. In orthopedics, VP finds most often used in nonimplant applications.
Powder bed fusion
PBF of polymers has the distinct advantages of both being able to fabricate complex structures and having a large library of biocompatible thermoplastics. However, a limiting factor of PBF is that it requires that the polymers have a narrow set of thermal properties to print viably. The most common variety of PBF printing for polymers is SLS. For nondegradable polymers, the material that is by far the most commonly used for PBF is polyamides, making up more than 95% of the current PBF market, with PA12 and PA11 being the most common varieties.
In SLS printing, the major build parameters are laser power, bed temperature, layer thickness, scan spacing, scan speed, and part raster pattern. Laser power, scan spacing, and scan speed are often bundled and expressed as the amount of energy delivered by the laser to the polymer powder. Appropriate energy delivery by laser and bed heating is important for printing serviceable parts. Powder properties are also well documented to be crucial to print quality, which are primarily affected by powder morphology. In addition, environmental effects such as humidity and electrostatic charging should also be taken into account. , Another important consideration in polymer PBF is powder aging and reuse. The ability to reuse polymer powders from prior print runs improves the economy of PBF printing significantly. Powders that are used in a print run are subject to thermal aging and often result in parts with inferior print quality, but a mitigation approach that is frequently used is to mix virgin powders with aged powders to minimize the decrease in part quality. Postprinting powder removal is also important to consider.
In the context of orthopedics, polymer PBF is used in both implant and nonimplant applications, and currently it is the only printing approach that has been used for commercial orthopedic implants. For implant applications, PAEK polymers are a common choice because of their vast clinical usage history and good printed properties. Commercial PAEK devices include a suture anchor, craniofacial implants, and spinal implants. For nonimplant materials, a common choice is any nylon variety, with common applications in surgical guides. Although there is obviously much potential in the use of PBF for printing orthopedic devices, currently there are relatively few commercially available powders that are optimized for PBF, with the majority of them being nonimplant grade.
Inkjet
Inkjet (IJ) printing is considered to be the most complex yet most accurate printing method, resulting in multicolor parts with a good finish. However, the printed parts are mechanically weaker than standard injection-molded parts. Material availability is also very limited compared to other printing methods because of stringent property requirements, and commercial resins are typically proprietary and machine specific. IJ can be classified into MJ and BJ. Both use photopolymers, although BJ processes can also use other substances for binding, such as solvents. Some processing considerations of IJ are similar to VP, wherein the photopolymer needs to cure properly during the build process. A crucial difference to VP is the need to consider the jetting process. Additionally, for BJ there is also a need to take powder properties into account. Postprint powder removal is also important in medical applications. In medical devices and orthopedics specifically, IJ is most often used for planning and visualization aids.
Summary and future trends
A summary of some of the polymers described in this section is presented in Tables 3.1 to 3.3 , for thermoplastics, thermosets, and photopolymers, respectively. Polymer data is available in different grades, specifications, and testing methods. The data presented in the table is meant to be used only as a broad guidance. Typical orthopedic applications of the polymer as a nondegradable are also shown. To note is that photopolymer resins used in commercial 3D printing are typically sold under trade names (e.g., VeroBlack [Stratasys], Accura [3D Systems], etc.), and their exact compositions are not publicly released. These polymers are presented under their trade names in the summary table.
Material | Common Print Method(s) | Mechanical Properties | Applications in Orthopedics | |
---|---|---|---|---|
Polyamide (nylon 66) | Extrusion, PBF | UTS | 85 MPa |
|
Mod | 2.0 GPa | |||
Failure strain | 300% | |||
PEEK | Extrusion, PBF | UTS | 95 MPa |
|
Mod | 6.5 GPa | |||
Failure strain | 3.5% | |||
PEKK | Extrusion, PBF | UTS | 73 MPa |
|
Mod | 3.3 GPa | |||
Failure strain | 13.2% | |||
ABS | Extrusion | UTS | 57 MPa |
|
Mod | 2.6 GPa | |||
Failure strain | 50% | |||
PLA | Extrusion | UTS | 65 MPa |
|
Mod | 2.5 GPa | |||
Failure strain | 65% | |||
UHMWPE | PBF | UTS | 40 MPa |
|
Mod | 0.8 GPa | |||
Failure strain | 350% | |||
PMMA | Extrusion, PBF | UTS | 76 MPa |
|
Mod | 3.1 GPa | |||
Failure strain | 10% | |||
PP | Extrusion, PBF | UTS | 30 MPa |
|
Mod | 1.7 GPa | |||
Failure strain | 140% | |||
PCU | Extrusion, | UTS | 50 MPa |
|
Mod | 0.08 GPa | |||
Failure strain | 400% | |||
PSU | Extrusion | UTS | 87 MPa |
|
Mod | 5.0 GPa | |||
Failure strain | 28% |
Material | Common Print Method(s) | Mechanical Properties | Applications in Orthopedics | |
---|---|---|---|---|
Polydim-ethylsiloxane | Extrusion, MJ | UTS | 2 MPa |
|
Mod | 360 MPa | |||
Failure strain | 300% |
Material | Common Print Method(s) | Mechanical Properties | Applications in Orthopedics | |
---|---|---|---|---|
Durable (Formlabs) | VP | UTS | 28 MPa |
|
Mod | 1 GPa | |||
Failure strain | 55% | |||
Accura PEAK (3D Systems) | VP | UTS | 67 MPa | |
Mod | 4.5 GPa | |||
Failure strain | 2% | |||
RPU 60 (Carbon) | VP | UTS | 48 MPa | |
Mod | 1.6 GPa | |||
Failure strain | 130% | |||
VisiJet M3-X (3D Systems) | MJ | UTS | 50 MPa | |
Mod | 2.1 GPa | |||
Failure strain | 8.3% | |||
VisiJet SR200 (3D Systems) | MJ | UTS | 34 MPa | |
Mod | 1.7 GPa | |||
Failure strain | 7.3% | |||
VisiJet PXL | BJ | UTS | 14 MPa | |
Mod | 9.4 GPa | |||
Failure strain | 0.23% |
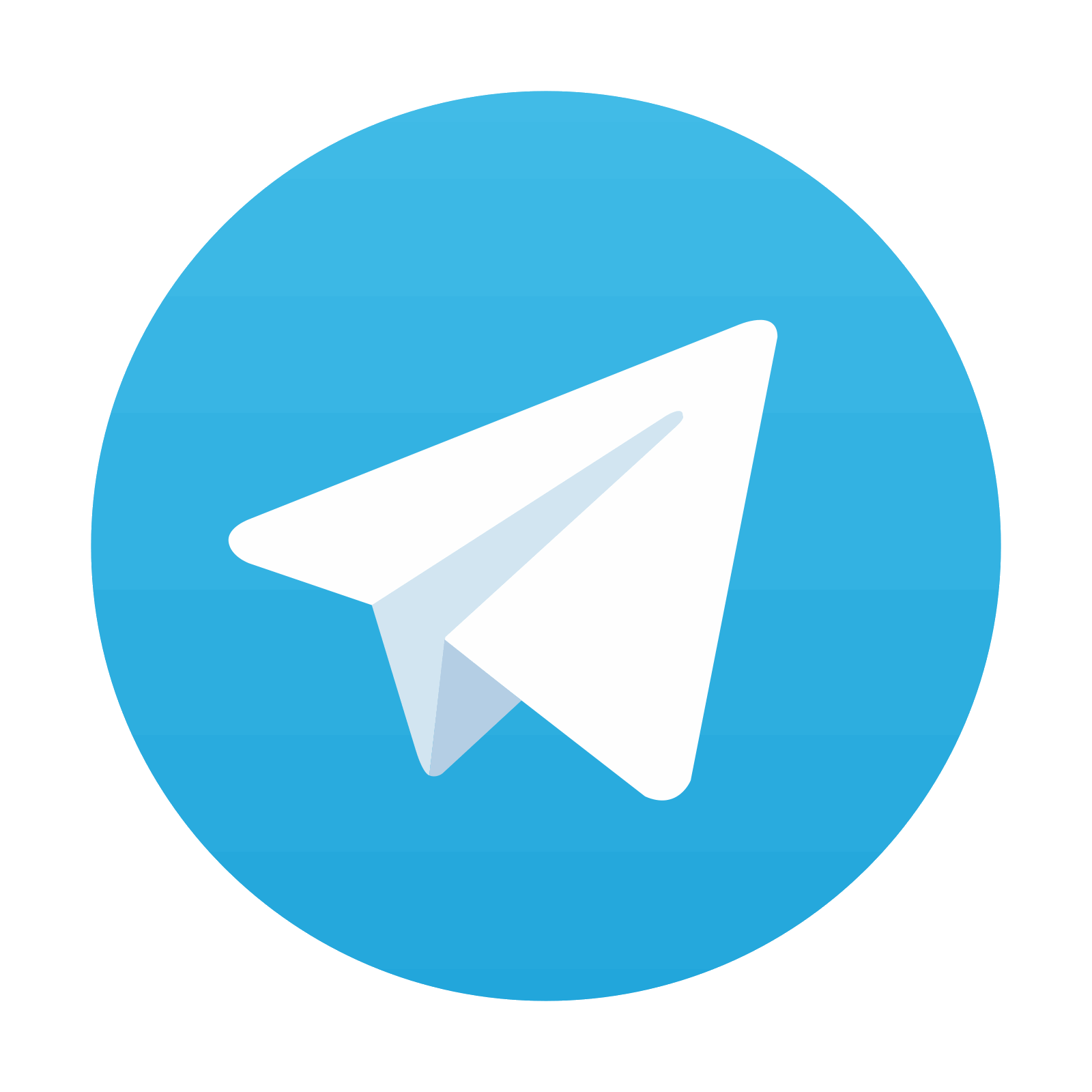
Stay updated, free articles. Join our Telegram channel
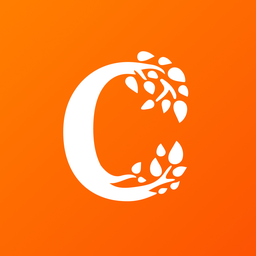
Full access? Get Clinical Tree
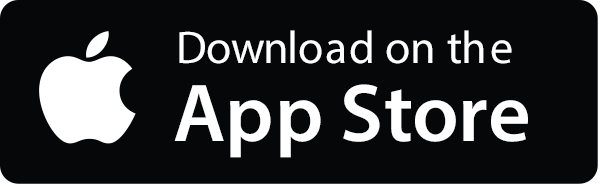
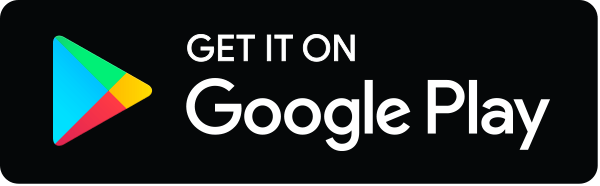
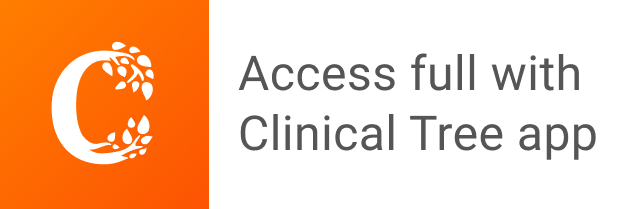