Introduction
3D printing has changed the landscape of medical devices by accelerating innovation and expanding what is possible to make. Ideas that used to be too expensive or not possible can now be easily 3D printed. Today 3D printing is used for several applications, ranging from toys to complex surgical implants. While 3D printing has been applied to many disciplines, this chapter will focus on concepts relevant to orthopedic surgery. This chapter will provide a framework for understanding 3D printing concepts that can be used to take a deep dive and apply principles in more specific topics in subsequent chapters. The chapter will start by introducing the advantages of 3D printing and outlining the historical development of 3D printing, before introducing the various methods of 3D printing and explaining how 3D printing technology is regulated by the US Food and Drug Administration (FDA).
Advantages of additive manufacturing
Additive manufacturing (AM) is an overarching term to describe a process of selectively joining material to create a 3D object. In contrast, traditional manufacturing methods, such as subtractive manufacturing, involve selectively removing parts of the starting material to create the final object. AM is commonly referred to as “3D printing,” and the two terms are often used interchangeably. Despite AM technology being relatively new, it has revolutionized manufacturing since its inception in the 1980s. The rapid adoption of AM is due to the many advantages AM has over traditional manufacturing, which are important to keep in mind when deciding if AM is the correct approach for a specific application.
Economically produce custom items
The production of custom items without AM techniques is time and cost prohibitive. Creating custom metal parts is a prime example. Technical expertise is needed to accurately machine metal parts to the desired specifications. With AM, a new 3D model can be created using computer-aided design (CAD) and fabricated with a 3D printer. Most manufacturing methods follow the economy of scale, where the unit cost decreases as the total number of units increases. However, with 3D printing the cost per unit does not change significantly between 1 and 100 units. The ability to practically produce custom parts has been leveraged in orthopedic device development to use a patient’s imaging to create a device that matches to the patient’s unique anatomy.
Rapid prototyping
The ability to easily create custom objects has accelerated innovation. Before the advent of AM, prototyping was expensive, and it took a long time to create the prototype. This bottleneck would limit the number of prototypes designers would choose to create. Using AM, developers can now quickly and cheaply iterate through several designs. They can quickly test what works well and what does not, therefore increasing the speed to market and quality of devices.
Complex structures
AM allows for the fabrication of structures that are either not practical or not possible using traditional manufacturing methods. For example, curved structures that are expensive to create by machining are just as easy to create as straight structures when using AM. AM also allows engineers to precisely introduce and control internal structures like porosity. In comparison, conventional methods to create porous structures, such as salt leaching, do not allow precise control of the size and distribution of the pores. The ability to create porous structures has been used to create implantable devices where the surrounding tissue, such as bone, can grow into the device and effectively integrate the device into the body.
Less wasted material
AM processes generally use fewer materials than subtractive manufacturing methods. Subtractive manufacturing methods involve starting with a block of material larger than the final part and removing the excess material. The excess materials can either be expensive to reuse (e.g., metal scraps in machining), or not possible to reuse (e.g., wood shavings in carpentry). In contrast, some AM methods only deposit material that is used for the final part, while other AM methods allow for excess material to be reused. The reduction in wasted materials can reduce the cost and environmental impact of AM parts.
Historical perspective of 3D printing
The development of 3D printing has rapidly expanded since the 1980s. Early versions of 3D printers were limited to specific materials and prohibitively expensive. Today’s 3D printers are used by everyone from hobbyists making toys to medical manufacturers creating implants. The following section will outline key milestones in 3D printing. A historical perspective on 3D printing will highlight the pace and scope of past innovation and establish the trajectory for the future.
Foundation of 3D printing techniques
The underlying process for the first 3D printer was proposed in 1977 when Swainson and Kramer outlined a process to create 3D structures by using light to selectively polymerize photoactive resin. This process would later become known as stereolithography (SLA). Three years later, Hideo Kodama filed a provisional patent for a light-based 3D printing process, but he did not file the full application in time to be granted a full patent. Hideo Kodama’s contributions to the development of 3D printing were documented in 1981 when he published the first article demonstrating 3D printing entitled “Automatic Method for Fabricating a Three-Dimensional Plastic Model With Photo-Hardening Polymer” ( Fig. 1.1 ).

The first full patent for an SLA printer was issued to Chuck Hall in 1986. In his patent application, he described the printer as:
A system for generating three-dimensional objects by creating a cross-sectional pattern of the object to be formed at a selected surface of a fluid medium capable of altering its physical state in response to appropriate synergistic stimulation by impinging radiation, particle bombardment or chemical reaction, successive adjacent laminae, representing corresponding successive adjacent cross-sections of the object, being automatically formed and integrated together to provide a step-wise laminar buildup of the desired object, whereby a three-dimensional object is formed and drawn from a substantially planar surface of the fluid medium during the forming process .
In other words, Chuck Hall described a method whereby a liquid starting material is turned into a 3D object by selectively exposing the liquid to radiation or a specific reactant to solidify a 2D plane, one layer at a time. He went on to cofound 3D Systems, which brought the first commercial SLA printer to market in 1988. 3D Systems continues to be an important contributor in the 3D printing space to date. For his work on SLA, the STL (STereoLithograph) format commonly used for 3D files, and 3D Systems, Chuck Hall is considered by many to be the father of 3D printing.
The development of SLA printing inspired the invention of other 3D printing methods that are commonplace today. Carl Deckard at the University of Texas applied for the first patent describing selective laser sintering (SLS) in 1986 and was granted the full patent in 1989. The patent described SLS as:
An apparatus for selectively sintering a layer of powder to produce a part made from a plurality of sintered layers .
In SLS, powder particles are selectively fused together to form the final 3D object, and SLS was the first example of powder bed fusion (PBF). That same year, Scott Crump applied for a patent for an:
apparatus incorporating a movable dispensing head provided with a supply of material which solidifies at a predetermined temperature, and a base member, which are moved relative to each other along “X,” “Y,” and “Z” axes in a predetermined pattern to create three-dimensional objects by building up material discharged from the dispensing head onto the base member at a controlled rate .
He described a method where a material is melted and extruded out of a nozzle that can move in the three spatial directions (x, y, and z), where it solidifies into a 3D object. The process described by the patent is the foundation for fused deposition modeling (FDM), the form of 3D printing most familiar to people today. Scott Crump went on to cofound Stratasys, which remains a key player in the 3D printing market today. Also occurring in 1989, Emanual Sachs at MIT submitted a patent for:
A process for making a component by depositing a first layer of a fluent porous material, such as a powder, in a confined region and then depositing a binder material to selected regions of the layer of powder material to produce a layer of bonded powder material at the selected regions.
In the new printing process, a binder solution is selectively deposited onto a powder bed to produce a 3D object. Sachs’ publication of the new printing method marked the beginning of the term “3D printing” being used to describe AM and was the foundation for binder jet printing. Z Corporation commercialized binder printing in 1993 and was later acquired by 3D Systems in 2012. Shortly after binder printing was commercialized, a new kind of printing was induced when Heisley Cooperation received a patent in 1999 for a new method of 3D printing invented by Michael Feygin for:
A laminated object manufacturing (LOM) system for forming a plurality of laminations into a stack to create a three-dimensional object.
By stacking and laminating cut-out sheets of materials, a 3D object can be formed relatively quickly.
The early pioneers of 3D printing described here the laid the foundation for the field. Thanks to their work, 3D printing was poised to rapidly evolve to meet the needs of diverse fields. The choice of materials, scale, speed, precision, and cost of 3D printing quickly improved throughout the 21st century and allowed for the applications of 3D printing to quickly expand beyond research labs.
3D printing in medicine
The newfound ability to fabricate patient-specific parts rapidly and cheaply led to 3D printing quickly establishing its foothold in medicine. Scientists have continued to apply 3D printing techniques to develop educational models, surgical guides, prosthetics, implants, and artificial organs. Early adoption of medical 3D printing was led by dentistry, orthopedic surgery, and urology. As early as 1994, 3D printing was reported to be used to plan reconstructive craniofacial surgery. ,
3D-printed implants
The ability to 3D print high-strength biocompatible materials opened the door to printed weight-bearing structures. An early example occurred in 2008 with the first 3D-printed leg prosthetics. Shortly afterward, the world saw the first reported upper extremity prosthetic: in 2015, a carpenter lost his fingers in an accident and his treatment spurred a global collaboration led by Ivan Owen, a mechanical special effects artist.
The 2010s saw a wave of FDA-cleared orthopedic implants ( Fig. 1.2 ). In contrast to prosthetics, implantable devices require more rigorous evaluation and control processes to be utilized. In 2010 Exactech received FDA clearance for the first 3D-printed orthopedic implant. The Novation Crown Cup with InteGrip is an acetabular cup with a porous surface created with AM techniques ( Fig. 1.3 ). The vast majority of the approved printed devices (79%) from 2000 to 2016 were made of metal. The first polymer implant was not approved until 2013 when Oxford Performance Material developed OsteoFab Patient-Specific Facial Device (OPSFD), a patient-specific cranial implant printed using poly-ether-ketone-ketone (PEKK) ( Fig. 1.4 ). Oxford Performance Material expanded on their printing technology to develop the SpineFab Vertebral Body Replacement (VBR) System, the first polymeric spinal implant approved by the FDA in 2015 ( Fig. 1.5 ).




Bioprinting
Bioprinting is holistically defined as fabrication using “computer-aided transfer processes for patterning and assembling living and nonliving materials with a prescribed 2D or 3D organization in order to produce bioengineered structures serving in regenerative medicine, pharmacokinetic, and basic cell biology studies.” , Bioprinting technologies are commonly grouped into three distinctive categories relative to their fabrication methods and similar modalities. These groups include extrusion-based bioprinting, droplet-based bioprinting, and energy-based bioprinting ( Fig. 1.6 ). Early iterations of 3D printers were limited in material selection, resolution, and speed. As the technology evolved, scientists inched closer to being able to print fully functional tissues or organs. A critical breakthrough came in 2000 when Thomas Boland, a bioengineer at the University of Texas, introduced the field of bioprinting. He modified an HP inkjet printer to lay down a bioink composed of living cells suspended in culture media. Since then, tissue engineers have worked on improving bioprinting techniques to fabricate bone or cartilage constructs. By printing large segments of tissues with a patient’s own cells, providers can treat large defects without the drawbacks of autografts or allografts, including limited supply, donor site morbidity, need for intraoperative modifications, risk of immune rejection, and long-term mechanical failure. Bioprinted bone and cartilage constructs have been successful in cell and animals studies, but they are not commercially available to date. Limitations for the clinical application of bioprinting include small size of bioprinted constructs, limited number and diversity of cells in the construct, lack of robust vascular networks to support cell growth, relatively poor mechanical properties that are insufficient to withstand physiological loads, and a lack of clear regulatory guidance from the FDA specific to bioprinting. ,

Introduction to 3D printing technologies
Since the early establishment of SLA 3D printing, many AM methods have been developed ( Fig. 1.7 ). Each method has its pros and cons when it comes to material selection, resolution, speed, cost, mechanical properties, and the technical skills needed to operate the machine. The various methods are characterized into seven general groups according to the ASTM F2792 standard. Each method has its own advantages and disadvantages, which are important to understand to determine appropriate methods for specific applications ( Table 1.1 ). The following section will outline and provide a brief introduction to the seven groups.

Methods of 3D Printing | Principle | Subgroups | Common Materials | Advantages | Limitations |
---|---|---|---|---|---|
Vat photopolymerization | A basin of a liquid photopolymer is selectively exposed to light to solidify an object layer by layer |
| Photopolymers (e.g., methacrylates) |
|
|
Powder bed fusion | For each layer, a uniform layer of powder is added to the print bed. An energy source is used to selectively fuse powder to form a solid object |
|
|
|
|
Extrusion | The starting filament is heated and extruded through a nozzle that moves in the x, y, or z direction | FDM/FFF | Thermoplastic polymers (e.g., PLA, ABS, PCL, PCU) |
|
|
Binder jet | For each layer, a uniform layer of powder is added to the print bed and a liquid binder is selectively added to form a solid object |
|
|
| |
Sheet lamination | A thin sheet of material is cut into a specified shape and fused to adjacent layers |
|
|
|
|
Material jetting | Analogous to a 2D inkjet printer, material is jetted onto a build plate in a layer-wise fashion | Polyjetting |
|
|
|
Direct energy deposition | An energy source directly fuses material coming out of a nozzle that can move in the x, y, or z direction |
| Metals that can be welded (e.g., Ti, CoCr) | Fast print speed
|
|
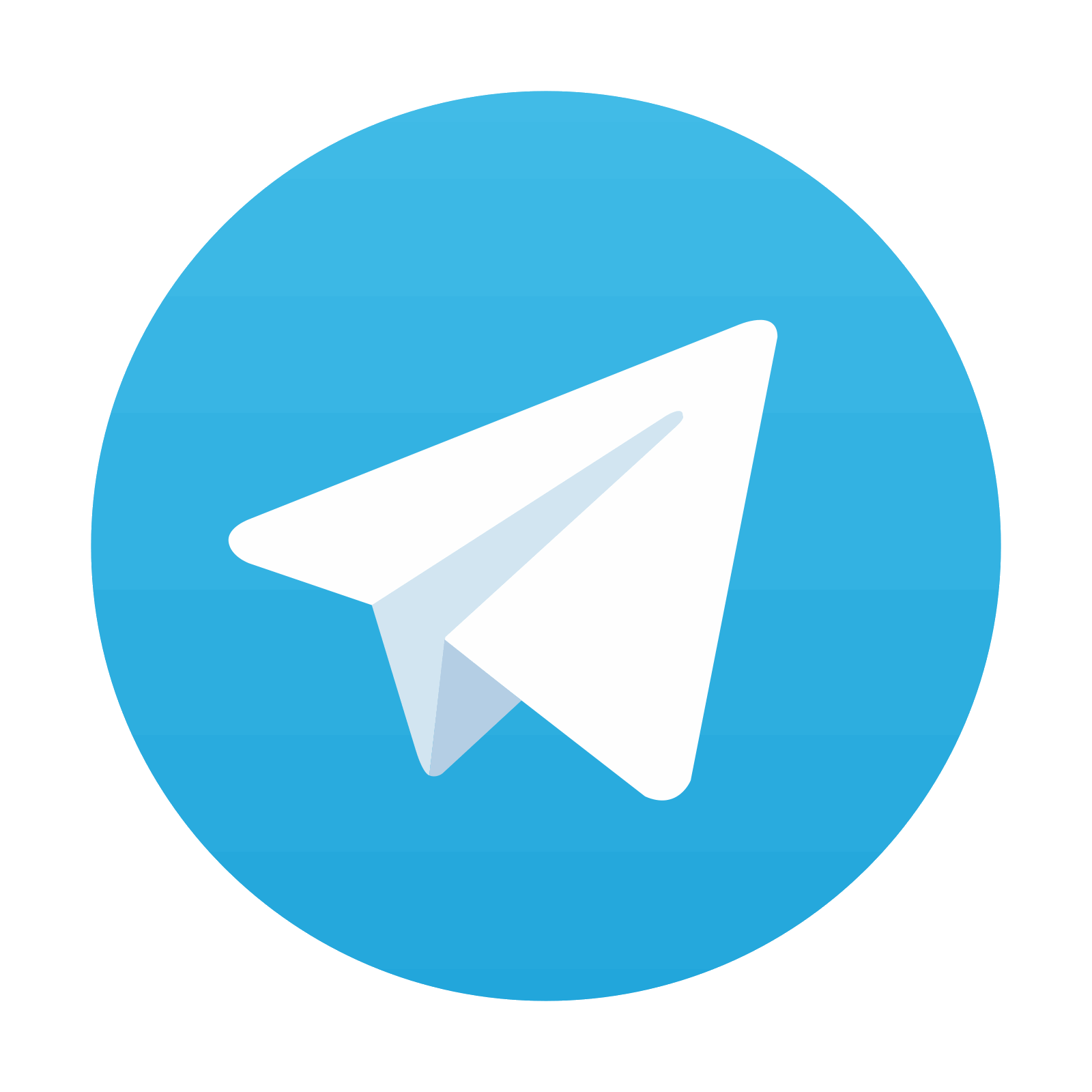
Stay updated, free articles. Join our Telegram channel
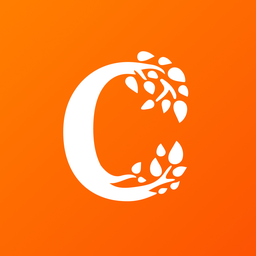
Full access? Get Clinical Tree
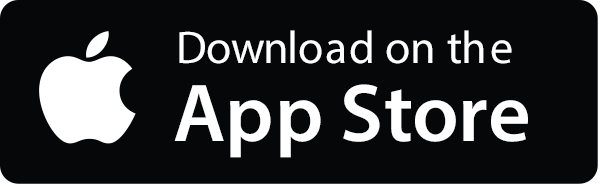
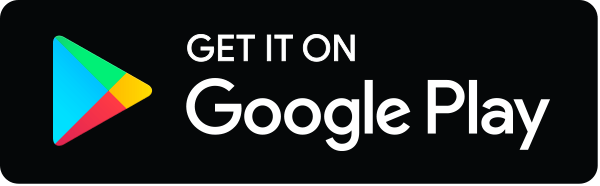
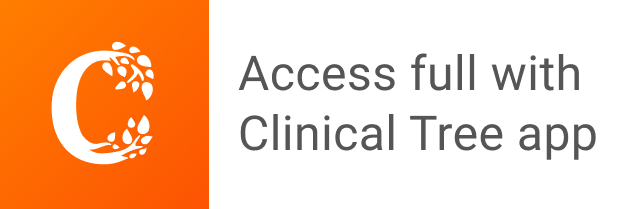