Maintaining Cardiorespiratory Fitness During Rehabilitation
Patrick Sells, DA, CES
William E. Prentice, PhD, PT, ATC, FNATA
After reading this chapter,
the athletic training student should be able to:
- Explain the relationships between heart rate, stroke volume, cardiac output, and rate of oxygen use.
- Describe the function of the heart, blood vessels, and lungs in oxygen transport.
- Describe the oxygen transport system and the concept of maximal rate of oxygen use.
- Describe the principles of endurance and high-intensity interval training and the potential of each technique for improving aerobic activity.
- Describe the difference between aerobic and anaerobic activity.
- Describe the principles of reversibility and detraining.
- Describe caloric threshold goals associated with various stages of exercise programming.
Although strength and flexibility are commonly regarded as essential components in any injury rehabilitation program, often relatively little consideration is given toward maintaining aerobic capacity and cardiorespiratory endurance. When musculoskeletal injury occurs, the patient is forced to decrease physical activity, and levels of cardiorespiratory endurance may decrease rapidly. Thus, the athletic trainer must design or substitute alternative activities that allow the individual to maintain existing levels of aerobic capacity during the rehabilitation period. Furthermore, the importance of maintaining and improving functional capacity is becoming increasingly evident regardless of musculoskeletal injury.15 Recent research has shown that increased levels of physical activity reduces the risk of cardiovascular disease.1 Sandvik et al37 reported mortality rates according to fitness quartiles during 16 years of follow-up. The number of deaths in the least-fit portion of the study outnumbered the deaths of the most fit by a margin of 61 to 11 deaths from cardiovascular causes.37 Myers et al studied 6213 patients referred for treadmill testing and concluded that exercise capacity is a more powerful predictor of mortality among men than other established risk factors for cardiovascular disease.28
By definition, cardiorespiratory endurance is the ability to perform whole-body activities for extended periods without undue fatigue.9 The cardiorespiratory system provides a means by which oxygen is supplied to the various tissues of the body. Without oxygen, the cells within the human body cannot function and, ultimately, cell death will occur. Thus, the cardiorespiratory system is the basic life-support system of the body.9,23
TRAINING EFFECTS ON THE CARDIORESPIRATORY SYSTEM
Basically, transport of oxygen throughout the body involves the coordinated function of 4 components: heart, blood vessels, blood, and lungs. The improvement of cardiorespiratory endurance through training occurs because of increased capability of each of these 4 elements in providing necessary oxygen to the working tissues.36,40 A basic discussion of the training effects and response to exercise that occur in the heart, blood vessels, blood, and lungs should make it easier to understand why the training techniques discussed later are effective in improving cardiorespiratory endurance.
Clinical Decision-Making Exercise 10-1
A freshman goalie on the soccer team is not very fit. The coach wants to get her started on a training program to improve her cardiorespiratory endurance. What principles should be considered when designing her program?
Adaptation of the Heart to Exercise
The heart is the main pumping mechanism and circulates oxygenated blood throughout the vascular system to the working tissues. The heart receives deoxygenated blood from the venous system and then pumps the blood through the pulmonary vessels to the lungs, where carbon dioxide is exchanged for oxygen. The oxygenated blood then returns to the left atrium of the heart, into the left ventricle, from which it exits through the aorta to the arterial system, and is circulated throughout the body, supplying oxygen to the tissues.
Heart Rate
As the body begins to exercise, the working tissues require an increased supply of oxygen (via transport on red blood cells) to meet the increased metabolic demand (cardiac output). The working tissues use the decreasing concentration of oxygen as a signal to vasodilate the blood vessels in the tissue. This decreases the resistance to blood flow and allows for a decreased velocity of flow, thereby increasing O2 extraction.38 Increases in heart rate occur as one response to meet the demand. The heart is capable of adapting to this increased demand through several mechanisms. Heart rate shows a gradual adaptation to an increased workload by increasing proportionally to the intensity of the exercise and will plateau at a given level after about 2 to 3 minutes36 (Figure 10-1). Increases in heart rate produced by exercise are met by a decrease in diastolic filling time. Heart rate parameters change with age, body position, type of exercise, cardiovascular disease, heat and humidity, medications, and blood volume. Conditions that exist in any patient should be taken into consideration when prescribing exercise to improve aerobic endurance. The commonly used equation to predict maximal heart rate (MHR) is 211 – (0.64 × age) with no evidence of effects with regard to gender, physical activity levels, VO2max level, or BMI groups.29 Monitoring heart rate is an indirect method of estimating oxygen consumption.33 Additionally, any medications should be considered prior to assessment or evaluation of heart rate response. For example, patients taking beta blockers will have an attenuated heart rate response to exercise. In general, heart rate and oxygen consumption have a linear relationship with exercise intensity. The greater the intensity of the exercise, the higher the heart rate. This relationship is least consistent at very low and very high intensities of exercise (Figure 10-2). During higher-intensity activities, MHR may be achieved before maximum oxygen consumption, which can continue to rise despite reaching an age-predicted heart rate.25 Because of these existing relationships, it should be apparent that the rate of oxygen consumption can be estimated by monitoring the heart rate.3
Figure 10-1. Plateau heart rate. For the heart rate to plateau at a given level, 2 to 3 minutes are required.
Stroke Volume
A second mechanism by which the cardiovascular system is able to adapt to increased demands of cardiac output during exercise is to increase stroke volume (the volume of blood being pumped out with each beat).36 Stroke volume is equal to the difference between end diastolic volume and end systolic volume. Typical values for stroke volume range from 60 to 100 mL/beat at rest and 100 to 120 mL/beat at maximum.13 Stroke volume will continue to increase only to the point at which diastolic filling time is simply too short to allow adequate filling. This occurs at about 40% to 50% of maximal aerobic capacity, or at a heart rate of 110 to 120 beats/min. Above this level, increases in the cardiac output are accounted for by increases in heart rate (Figure 10-3).13
Figure 10-2. MHR is achieved at about the same time as maximal aerobic capacity.
Figure 10-3. Stroke volume plateaus at about 40% of MHR.
Cardiac Output
Stroke volume and heart rate collectively determine the volume of blood being pumped through the heart in a given unit of time. About 5 L of blood are pumped through the heart during each minute at rest. This is referred to as the cardiac output, which indicates how much blood the heart is capable of pumping in exactly 1 minute. Thus, cardiac output is the primary determinant of the maximal rate of oxygen consumption possible (Figure 10-4). During exercise, cardiac output increases to about 4 times that experienced during rest (to about 20 L) in the normal individual, and may increase as much as 6 times in the elite endurance athlete (to about 31 L).
Cardiac output = stroke volume × heart rate
Figure 10-4. Cardiac output limits maximal aerobic capacity.
This equation illustrates that any factor that will impact heart rate or stroke volume can either increase or decrease cardiac output. For example, an increase in venous return of blood from working muscle will increase the end diastolic volume. This increased volume will increase stroke volume via the Frank Starling mechanism39 and, therefore, cardiac output.45 Heart rate is regulated by the autonomic nervous system as well as circulating levels of epinephrine secreted from the adrenal medulla. Conversely, conditions that resist ventricular outflow (high blood pressure or an increase in afterload) will result in a decrease in cardiac output. Conversely, a condition that would decrease venous return (peripheral artery disease) would decrease stroke volume and attenuate cardiac output. Figure 10-5 outlines the factors that regulate both stroke volume and heart rate.
A commonly reported benefit of aerobic conditioning is a reduced resting heart rate and a reduced heart rate at a standard exercise load. This reduction in heart rate is explained by an increase in stroke volume brought about by increased venous return and to increased contractile conditions in the myocardium. The heart becomes more efficient because it is capable of pumping more blood with each stroke. Because the heart is a muscle, it can hypertrophy, or increase in size and strength as a result of aerobic exercise, to some extent, but this is in no way a negative effect of training.40
Training Effect
Increased stroke volume × decreased heart rate = cardiac output
During exercise, females tend to have a 5% to 10% higher cardiac output than males at all intensities. This is likely the result of a lower concentration of hemoglobin in the female, which is compensated for during exercise by an increased cardiac output.47
Adaptation in Blood Flow
The amount of blood flowing to the various organs increases during exercise. However, there is a change in overall distribution of cardiac output: the percentage of total cardiac output to the nonessential organs is decreased, whereas it is increased to active skeletal muscle.
Volume of blood flow to the heart muscle or myocardium increases substantially during exercise, even though the percentage of total cardiac output supplying the heart muscle remains unchanged. The increase in flow to skeletal muscle is brought about by withdrawal of sympathetic stimulation to arterioles, and vasodilation is maintained by intrinsic metabolic control.39 Trained persons have a higher capillary density than their untrained counterparts to better accommodate the increased supply and demand. In skeletal muscle, there is increased formation of blood vessels or capillaries, although it is not clear whether new ones form or dormant ones simply open up and fill with blood.39
The total peripheral resistance (TPR) is the sum of all forces that resist blood flow within the vascular system. TPR decreases during exercise primarily because of vessel vasodilation in the active skeletal muscles.
Figure 10-5. The factors effecting cardiac output.
Adaptation in Blood Pressure
Blood pressure in the arterial system is determined by the cardiac output in relation to TPR to blood flow as follows:
BP = CO × TPR
where BP = blood pressure, CO = cardiac output, and TPR = total peripheral resistance
Blood pressure is created by contraction of the myocardium. Contraction of the ventricles of the heart creates systolic pressure, and relaxation of the heart creates diastolic pressure. Blood pressure is regulated centrally by neural activity on peripheral arterioles and locally by metabolites produced during exercise. During exercise, there is a decrease in TPR (via decreased vasoconstriction) and an increase in cardiac output. Systolic pressure increases in proportion to oxygen consumption and cardiac output, whereas diastolic pressure shows little or no increase.33 Failure of systolic pressure to increase with increased exercise intensity is considered an abnormal response to exercise and is a general indication to stop an exercise test or session.1 Blood pressure falls below preexercise levels after exercise and may stay low for several hours. There is general agreement that engaging in consistent aerobic exercise will produce modest reductions in both systolic and diastolic blood pressure at rest as well as during submaximal exercise.10,30
Adaptations in the Blood
Oxygen is transported throughout the system bound to hemoglobin. Found in red blood cells, hemoglobin is an iron-containing protein that has the capability of easily accepting or giving up molecules of oxygen as needed. Training for improvement of cardiorespiratory endurance produces an increase in total blood volume, with a corresponding increase in the amount of hemoglobin. The concentration of hemoglobin in circulating blood does not change with training; it may actually decrease slightly.
Adaptation of the Lungs
As a result of training, pulmonary function is improved in the trained individual relative to the untrained individual. The volume of air that can be inspired in a single maximal ventilation is increased. The diffusing capacity of the lungs is also increased, facilitating the exchange of oxygen and carbon dioxide. Pulmonary resistance to air flow is also decreased. Both endurance training and high-intensity interval training are effective in increasing inspiratory muscle strength, while high-intensity interval training is more time-efficient in improving aerobic capacity and performance.12
Figure 10-6. Maximal aerobic capacity required during activity: The greater the percentage of maximal aerobic capacity required during an activity, the less time that activity can be performed.
Clinical Decision-Making Exercise 10-2
A lacrosse player sustained a season-ending knee injury at the end of last season. During the off-season, he began training for his return to hockey. After several months of training, what physiological changes should be occurring?
MAXIMAL AEROBIC CAPACITY
The maximal amount of oxygen that can be used during exercise is referred to as maximal aerobic capacity (exercise physiologists refer to this as VO2max). It is considered to be the best indicator of the level of cardiorespiratory endurance.44 Maximal aerobic capacity is most often presented in terms of the volume of oxygen used relative to body weight/unit of time (mL × kg-1 × min-1).4 A recently developed equation for predicting VO2max is as follows27:
VO2max (mL/kg-1/min-1) = 79.9 – (0.39 × age) – (13.7 × gender [0 = male; 1 = female]) – (0.127 × weight [lbs])
It is common to see aerobic capacity expressed in metabolic equivalents (METs). Resting oxygen consumption is generally considered to be 3.5 mL × kg-1 × min-1 or 1 MET. Therefore, an exercise intensity of 10 METs is equivalent to a VO2 of 35 mL × kg-1 × min-1. A normal maximal aerobic capacity for most collegiate men and women would fall in the range of 35 to 50 mL × kg-1 × min-1.18
Rate of Oxygen Consumption
The performance of any activity requires a certain rate of oxygen consumption, which is about the same for all persons, depending on their present level of fitness. Generally, the greater the rate or intensity of the performance of an activity, the greater will be the oxygen consumption. Each person has his or her own maximal rate of oxygen consumption. The person’s ability to perform an activity is closely related to the amount of oxygen required by that activity. This ability is limited by the maximal amount of oxygen the person is capable of delivering into the lungs. Fatigue occurs when insufficient oxygen is supplied to muscles. It should be apparent that the greater the percentage of maximal aerobic capacity required during an activity, the less time the activity may be performed (Figure 10-6).
Three factors determine the maximal rate at which oxygen can be used: (1) external respiration, involving the ventilatory process or pulmonary function; (2) gas transport, which is accomplished by the cardiovascular system (ie, the heart, blood vessels, and blood); and (3) internal (cellular) respiration, which involves the use of oxygen by the cells to produce energy. Exercise physiologists generally discuss the limiting factors of maximal aerobic capacity based on healthy human subjects in a controlled environment.5,11,30 Under these conditions, research presents agreement that the ability to transport oxygen through the heart, lungs, and blood is the limiting factor to the overall rate of oxygen consumption. This indicates that this is not the ability of the mitochondria to consume oxygen that limits VO2max. A high maximal aerobic capacity within a person’s range indicates that all 3 systems are working well.
Maximal Aerobic Capacity: An Inherited Characteristic
It has been suggested that the maximal rate at which oxygen can be used is a genetically determined characteristic.4 We inherit a certain range of maximal aerobic capacity, and the more active we are, the higher the existing maximal aerobic capacity will be within that range.38,46 Therefore, a training program is capable of increasing maximal aerobic capacity to its highest limit within our range.32,40,46
Fast- vs Slow-Twitch Muscle Fibers
The range of maximal aerobic capacity inherited is in a large part determined by the metabolic and functional properties of skeletal muscle fibers. As discussed in detail in Chapter 9, there are 3 distinct types of muscle fibers: slow-twitch and 2 variations of fast-twitch fibers, each of which has distinctive metabolic and contractile capabilities. Because they are relatively fatigue-resistant, slow-twitch fibers are associated primarily with long-duration, aerobic-type activities. The slow-twitch fibers depend on oxidative phosphorylation to generate adenosine triphosphate (ATP) to provide the energy needed for muscle contraction. Fast-twitch fibers are useful in short-term, high-intensity activities, which mainly involve the anaerobic system. Intermediated fast-twitch fibers demonstrate a reliance on glycolysis to produce ATP. These intermediate fibers also have the ability to adapt based on specific training regimens.23 In general, if a patient has a high ratio of slow- to fast-twitch muscle fibers, the patient will be able to use oxygen more efficiently and, thus, will have a higher maximal aerobic capacity.
Clinical Decision-Making Exercise 10-3
A cyclist wants to know if you can test his maximal aerobic capacity. He says that he has reached a plateau in his training. There hasn’t been an increase in his maximal aerobic capacity in about 1 year. What is your explanation for why this is occurring?
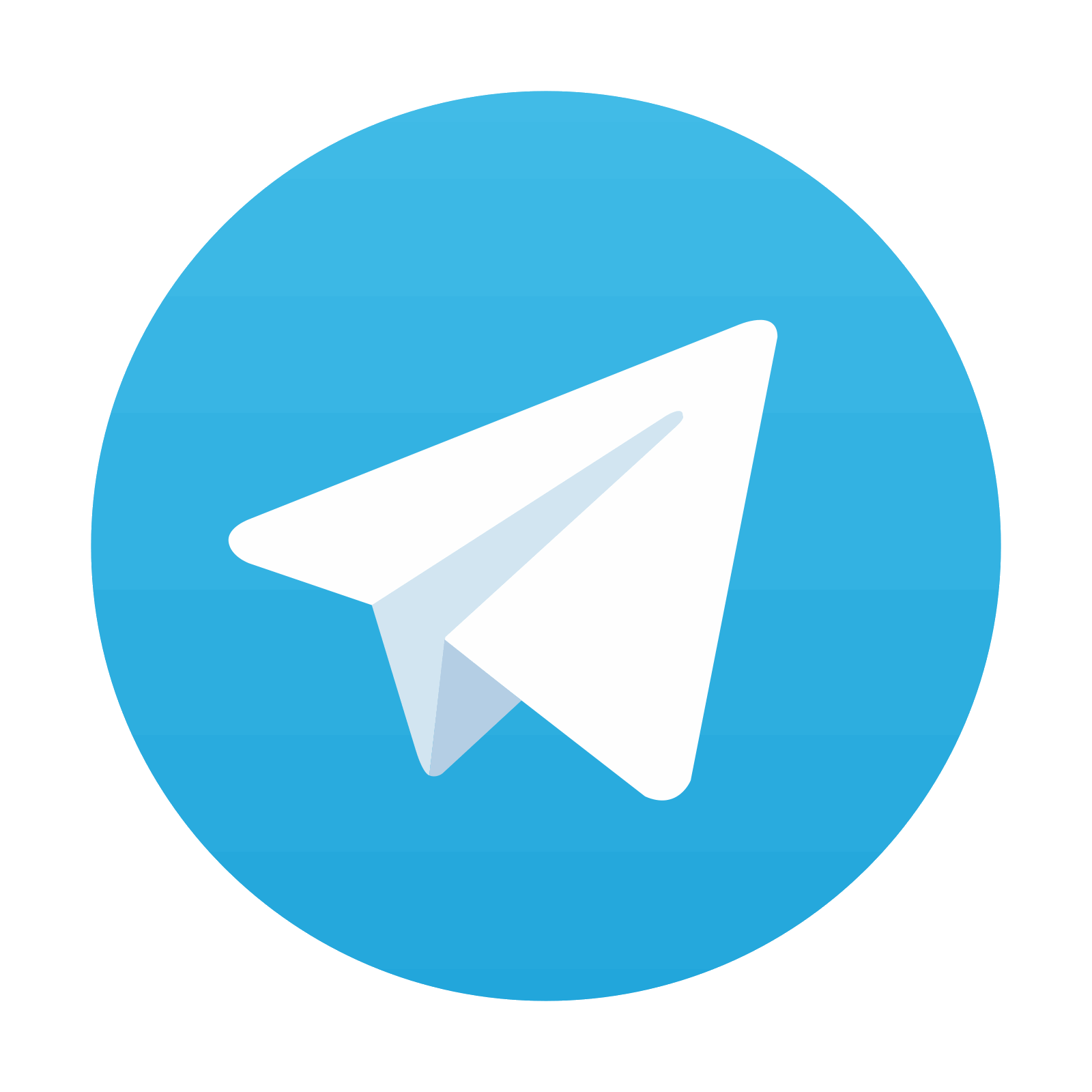
Stay updated, free articles. Join our Telegram channel
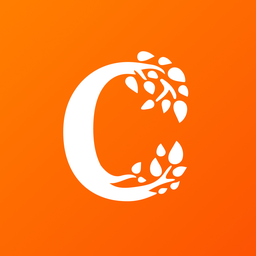
Full access? Get Clinical Tree
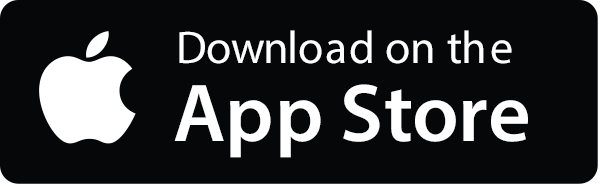
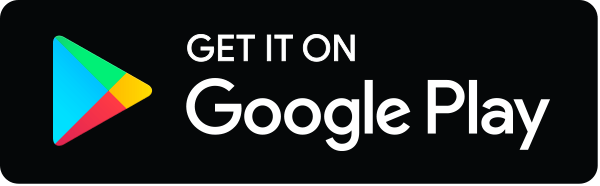