Magnetic Resonance Imaging of Cartilage Repair Techniques
The field of cartilage repair is expanding rapidly and encompasses a wide range of techniques, including microfracture, first- and second-generation autologous chondrocyte implantation, autologous osteochondral transplantation, and allograft transplantation. Although assessment of patient outcome is clinically relevant, objective evaluation of repair allows insight into the natural history of cartilage repair and may allow detection of early signs associated with a poorer prognosis. Objective assessment has traditionally been performed with second-look arthroscopy and histologic evaluation of biopsy specimens.1 Magnetic resonance (MR) imaging, however, offers a noninvasive method to assess cartilage repair. The information gained from MR imaging is therefore complementary to more subjective clinical outcome instruments that evaluate pain and function, and plays a valuable role in patient follow-up after cartilage repair.2
This chapter discusses the MR imaging techniques available for the assessment of articular cartilage, including advanced imaging techniques that allow assessment of cartilage biochemistry. The MR imaging appearance and assessment of microfracture, autologous chondrocyte implantation, and osteochondral autograft and allograft transplantation are reviewed.
Basic Structure of Articular Cartilage
An understanding of the structure of articular cartilage is crucial to understanding the MR imaging appearance of normal and abnormal cartilage, as well as the imaging appearance following cartilage repair. Articular cartilage is composed of chondrocytes that are embedded in an organized extracellular matrix composed primarily of water (65 to 80%), collagen, and proteolgycan.3 The material properties of articular cartilage are imparted mainly by the collagen and proteolgycan components of the extracellular matrix.
Compressive strength is imparted by the proteolgycan molecules, which are composed of negatively charged glycosaminoglycans radiating from a protein core.4 These monomers bind to hyaluronic acid to form large aggregates, which resist compression because of their hydrophilic nature. The structural framework and tensile strength of articular cartilage is imparted by collagen. Type II collagen fibers make up 95% of the collagen in articular cartilage. These fibers have a long length-to-thickness ratio, thereby providing tensile stiffness and strength.5
Articular cartilage can be divided into four distinct zones on histology, which can be depicted with cartilage-sensitive MR pulse sequences. The superficial zone and lamina splendens consist of highly organized collagen fibers oriented parallel to the cartilage surface, providing high tensile strength. The transitional zone has lower collagen content and consists of randomly oriented collagen fibers; this zone has a higher compressive strength than the superficial zone. The radial zone consists of highly organized collagen fibers oriented perpendicular to the cartilage surface; this zone has the highest proteolgycan content and the lowest water content. The deepest zone is the calcified cartilage layer. The tidemark represents the boundary between the uncalcified and calcified cartilage.6 At clinically relevant field strengths using traditional MR sequences, the tidemark is indistinguishable from the subchondral bone plate.
The signal characteristics of articular cartilage on MR imaging reflect both the mobility of water and the degree of organization of the tissue. Therefore, in the radial zone, where the collagen is highly ordered, lower signal intensity is generated when compared with the transitional zone where the collagen is more randomly oriented. The lamina splendens is also highly ordered and when visualized on MR images appears of lower signal intensity. It is important to recognize this normal “gray-scale stratification” when performing MR imaging of cartilage, as loss of the normal gray-scale stratification is one of the earliest signs of articular cartilage degeneration.6
Morphologic Assessment of Cartilage
Cartilage-Sensitive Pulse Sequences
Traditional T1- and T2-weighted techniques are inadequate for the accurate assessment of articular cartilage. T1-weighted images result in poor delineation between the intermediate signal intensity cartilage and the low- to intermediate-signal intensity joint fluid. Conventional spin-echo or heavily T2-weighed fast spin echo (FSE) techniques result in poor delineation between the subchondral bone and the deep component of the articular cartilage, with consequent factitious thickening of the subchondral bone and thinning of the articular cartilage.7 Several different cartilage-sensitive pulse sequences are available for MR imaging; of these, fat-suppressed three-dimensional (3D) gradient echo and FSE sequences are the most accurate and most widely used techniques.
Fat-suppressed 3D spoiled or T1-weighted gradient echo images obtained with isotropic voxels have the advantage of producing thin, contiguous slices that can be reformatted in any plane. The sharp contrast boundary between the low-signal intensity bone and the high-signal intensity articular cartilage makes these sequences amenable to semiautomated segmentation algorithms, allowing for accurate assessment of cartilage thickness and volume. This pulse sequence has been used for longitudinal assessment of cartilage volume in osteoarthritis trials and for quantitative assessment of focal cartilage defects and subsequent fill following repair.8–10 This technique is, however, less sensitive to partial-thickness cartilage defects than FSE sequences and requires longer scan times. It is also limited by metal-induced susceptibility artifact, which may be a significant problem when imaging in the presence of metallic hardware or residual metallic debris following arthroscopy.11
An intermediate echo time (TE) two-dimensional FSE technique is one of the most popular pulse sequences for the assessment of articular cartilage. This technique provides good contrast between the intermediate signal intensity of articular cartilage, the low signal intensity of fibrocartilage and subchondral bone, and the high signal intensity of synovial fluid. On intermediate TE FSE images, articular cartilage demonstrates a normal gray-scale stratification, which corresponds to the cartilage zonal anatomy. Partial-thickness chondral lesions and chondral flaps are also well depicted with this technique ( Fig. 2.1 ).12 Use of an FSE technique with a wide receiver bandwidth minimizes susceptibility artifact, allowing accurate assessment of cartilage in the presence of metallic hardware or debris.13
Intermediate TE FSE sequences are subject to the “magic angle” effect, which must be considered when interpreting these images. The signal intensity of ordered tissues, such as cartilage and tendons, depends on the orientation of the collagen fibers relative to the external magnetic field (B0), which in a conventional MR machine runs parallel to the long axis of the patient′s body. When highly structured tissues are imaged at 55 degrees to the external magnetic field using a short TE, there is a normal prolongation of T2 values, a phenomenon known as “magic angle” effect.14,15 In the knee, magic angle effect commonly manifests as increased signal intensity in the cartilage over the anterior and posterior femoral condyles and in the submeniscal zone of the posterior tibial condyles.

The application of fat suppression to FSE images allows the detection of bone marrow edema and increases the contrast differences between cartilage, fluid, and synovium. However, fat suppression results in a lower signal-to-noise ratio; therefore, it is more difficult to achieve the high spatial resolution that is required to discern subtle fissures and surface fibrillation in the articular cartilage.7,16 Thus, routine assessment of articular cartilage with FSE techniques should include high-resolution, non-fat-suppressed FSE images in at least two planes as well as a fat-suppressed FSE image in one plane.12
Evaluation of Cartilage Biochemistry
Quantitative MR imaging techniques allow more sophisticated assessment of cartilage degeneration and cartilage repair. These techniques detect changes in the ultrastructure of cartilage and provide an assessment of cartilage biochemistry. They therefore have the potential to detect changes in cartilage biochemistry that may precede discernible cartilage thinning on traditional MR techniques. Quantitative MR imaging techniques are classified into those that detect alterations in collagen fiber orientation and those that detect alterations in the proteoglycan content.
Collagen
T2 mapping is the most commonly used quantitative MR technique for the assessment of collagen orientation. Additional techniques such as diffusion tensor imaging have been shown to be sensitive to collagen orientation17–19 but are not currently in widespread clinical use.
T2 Mapping
The T2 (spin-spin) relaxation time reflects the loss of signal that occurs due to dephasing of the excited nuclei after the disturbing radio-frequency pulse is applied. T2 mapping is performed by acquiring several images at different TEs at the same slice location. The T2 calculation is performed on a pixel-by-pixel basis by fitting the signal intensity from each echo image and the corresponding TE to an exponential decay equation.
The T2 map of articular cartilage reflects the collagen fiber orientation and the mobile water content,20 and is displayed using a color-coded map. In the radial zone, where the collagen is oriented perpendicular to the subchondral plate, short T2 values are obtained. In the transitional zone, where there is a more random orientation of collagen, longer T2 values are obtained. Prolongation of T2 relaxation times has been shown to be associated with osteoarthritis and breakdown in cartilage structure.21–23 Quantitative T2 measurements demonstrate excellent inter- and intraobserver reliability,24–26 thereby offering a tool for reproducible assessment of cartilage status over time.
Proteoglycan
Imaging strategies aimed at assessing the proteoglycan component of the extracellular matrix exploit the fixed-charge density property of the articular cartilage. The fixed-charge density in cartilage is largely due to the concentration and distribution of the negatively charged glycosaminoglycan chains within the proteoglycan macromolecules. Techniques used to assess proteoglycan content and distribution include sodium-23 imaging, delayed gadolinium-enhanced MR imaging of cartilage (dGEMRIC), and T1rho (>)-weighted MR imaging.
Sodium Imaging
Similar to hydrogen nuclei, sodium-23 is also a suitable nucleus for MR imaging. The presence of the negatively charged glycosaminoglycan molecules in articular cartilage generates an attraction toward the positively charged sodium-23, allowing for relative measurement of fixed-charge density.27,28 Sodium imaging has been used as an imaging standard by which to assess proteoglycan distribution and content. However, this technique is limited in its clinical application by the lower concentration of sodium-23 in articular cartilage relative to hydrogen, resulting in low signal-to-noise ratio and requiring long scan times. Sodium imaging also requires the use of specialized coils and the ability to scan with multinuclear spectroscopy software, which is not widely available across clinical systems.29,30
dGEMRIC
dGEMRIC also exploits the fixed-charge property of articular cartilage through the use of an injection of negatively charged gadolinium contrast. Gadolinium is administered intravenously, the patient performs 10 minutes of exercise, and, following a 90-minute delay, T1-weighted maps are obtained, usually through the use of a specialized inversion recovery pulse sequence.31 The gadolinium penetrates the articular cartilage, with the amount of penetration being inversely proportional to the glycosaminoglycan content. The gadolinium acts to shorten T1-relaxation times, allowing for the generation of T1 maps. In areas with depleted glycosaminoglycan content, there will be an increased distribution of gadolinium and therefore a higher T1 signal, which is reflected by a diminished “relative glycosaminoglycan index.”
T1rho (ρ) Mapping
T1rho (ρ) is a technique used to assess the low-frequency interactions between hydrogen in macromolecules and free water. Termed spin-lattice relaxation in the rotating frame, this technique uses clusters of radio-frequency pulses to “lock” magnetization in the transverse plane and limit dephasing of protons. After a specified period of time (the spin-lock time), the magnetization vector is realigned with B0, and data are then acquired using an FSE or a spiral sequence.32,33 Similar to T2 mapping, T1rho is calculated on a pixel-by-pixel basis by fitting the signal intensity from each spin-lock image and the corresponding spin-lock length to an exponential decay equation.
T1rho has been shown to reflect proteoglycan content in articular cartilage. The normalized T1rho rate is highly correlated to fixed-charge density in sodium-23 imaging, as well as to proteoglycan content and distribution on histology.34–36 Subjects with osteoarthritis have longer T1rho values than asymptomatic controls, and T1rho may be even more sensitive to early cartilage degeneration than T2 mapping alone.37 While clinically feasible at 1.5T and 3T,37,38 T1rho is largely applied at 3T and is a promising technique to detect changes in proteoglycan content in early cartilage degeneration.
Due to the specialty coil requirements for sodium imaging as well as the logistical constraints of the contrast-enhanced dGEMRIC technique, the authors favor the use of non-contrast T1rho at 3T for assessment of proteoglycan content.
Evaluation of Articular Cartilage Repair Techniques
In part due to its limited vascular supply, mature articular cartilage has little to no capacity for spontaneous repair.39,40 A wide variety of cartilage repair techniques have been described; results following these procedures have varied widely. Most of the literature has relied on subjective clinical assessment or conventional radiographs to evaluate the success of repair techniques. The use of second-look arthroscopy with biopsy remains the gold standard but is limited by its invasive nature, potential for operative surgeon bias, and poor patient acceptance. With advancements in imaging techniques, MR imaging is increasingly recognized as an alternative method of noninvasive evaluation of the results of articular cartilage repair.41
The variables that should be assessed on MR imaging following cartilage repair differ according to the repair technique2 and are summarized in Table 2.1 . The expected MR imaging appearance following commonly used cartilage repair techniques is summarized in Table 2.2 .
Microfracture
|
Autologous chondrocyte implantation (ACI)
|
Osteochondral transplantation Osseous phase
Articular phase
Other features
|
Microfracture Early postoperative period
Late postoperative period
Specific complication
|
Autologous chondrocyte implantation (ACI) Early postoperative period
Late postoperative period
Specific complications
|
Osteochondral autografts Early postoperative period
Late postoperative period
Specific complications
|
Osteochondral allograft (OCA) Early postoperative period (0–3 mo)
Late postoperative period (3–6 mo)
Specific complications
|
Microfracture
Microfracture is a cartilage repair technique that is based on local bone marrow stimulation and the release of multipotential stem cells,42 which, over time, differentiate into repair tissue, predominantly composed of fibrocartilage.43,44
MR imaging assessment following micro-fracture should include the assessment of (1) the signal intensity of the repair cartilage compared with native cartilage; (2) the morphology of the repair, being either flush, proud, or depressed with respect to the native cartilage; (3) the volume or percent fill of the defect in two imaging planes; (4) the degree of peripheral integration to the adjacent cartilage, including the absence/presence of fissures; (5) assessment of the underlying bone, including the presence of bone marrow edema; (6) the surface geometry of the bone, including the presence of proud subchondral bone; (7) the status of the articular cartilage in the adjacent and opposing surfaces; and (8) the presence of a reactive synovitis.2
The MR imaging appearance of a chondral defect treated by microfracture evolves over time. In the early postoperative period, the reparative tissue is less organized and has increased water content when compared with normal articular cartilage, and as such, it appears hyperintense compared with the native cartilage.2,45 Over time, there is maturation of the signal characteristics of the repair tissue, which may appear hypointense to the native cartilage,2,45 suggesting the presence of reparative fibrocartilage.46–48
The percent fill of the defect increases with time; however, the overall percent fill following microfracture may be less than that seen following autologous chondrocyte implantation.2 Fissures between the area of microfracture and native cartilage appear commonly45 and do not necessarily correlate to symptoms.2 By 1 to 2 years after surgery, the treated defect should be filled with tissue that has a smooth, well-defined surface. Adverse functional scores at 2 years have been shown to correlate with poor percentage fill, indicating a correlation between objective MR imaging findings and subjective clinical outcome.45
Bone marrow edema may be seen in the subchondral bone at short-term follow-up but usually appears mild2 and gradually diminishes over time.44 In patients with failure of the microfracture procedure, the subchondral bone marrow edema does not diminish and may become more conspicuous over time.
Because microfracture involves the release of pluripotential stem cells, these cells may also differentiate into bone. Overgrowth of the subchondral bone has been noted in 25 to 49% of patients following microfracture2,45 ( Fig. 2.2 ). Osseous overgrowth may not be a negative prognostic factor and does not correlate with adverse clinical scores at short-term follow-up.45 Osseous overgrowth may, however, result in a thinner layer of reparative tissue and inferior filling of the cartilage defect, which has been shown to correlate to inferior functional outcomes.49
Studies using quantitative MR imaging of microfracture have shown that at short-term follow-up (< 6 months) T2 values over the repair site are prolonged, mimicking the signal characteristics of the repair tissue observed on conventional MR imaging. Over time (> 12 months), there is progressive shortening and maturation of T2 values,50 although T2 values of the repair tissue may never return to normal. At a follow-up time of 24 months after microfracture, T2 values have been shown to remain globally reduced compared with native cartilage.51,52 The T2 index, calculated by the T2 value of the repair tissue compared with native cartilage, correlates with subjective functional scores, such as the Lysholm score and International Knee Documentation Committee (IKDC) form of subjective function,51 further supporting the use of quantitative MR imaging as an objective tool to evaluate cartilage repair procedures.
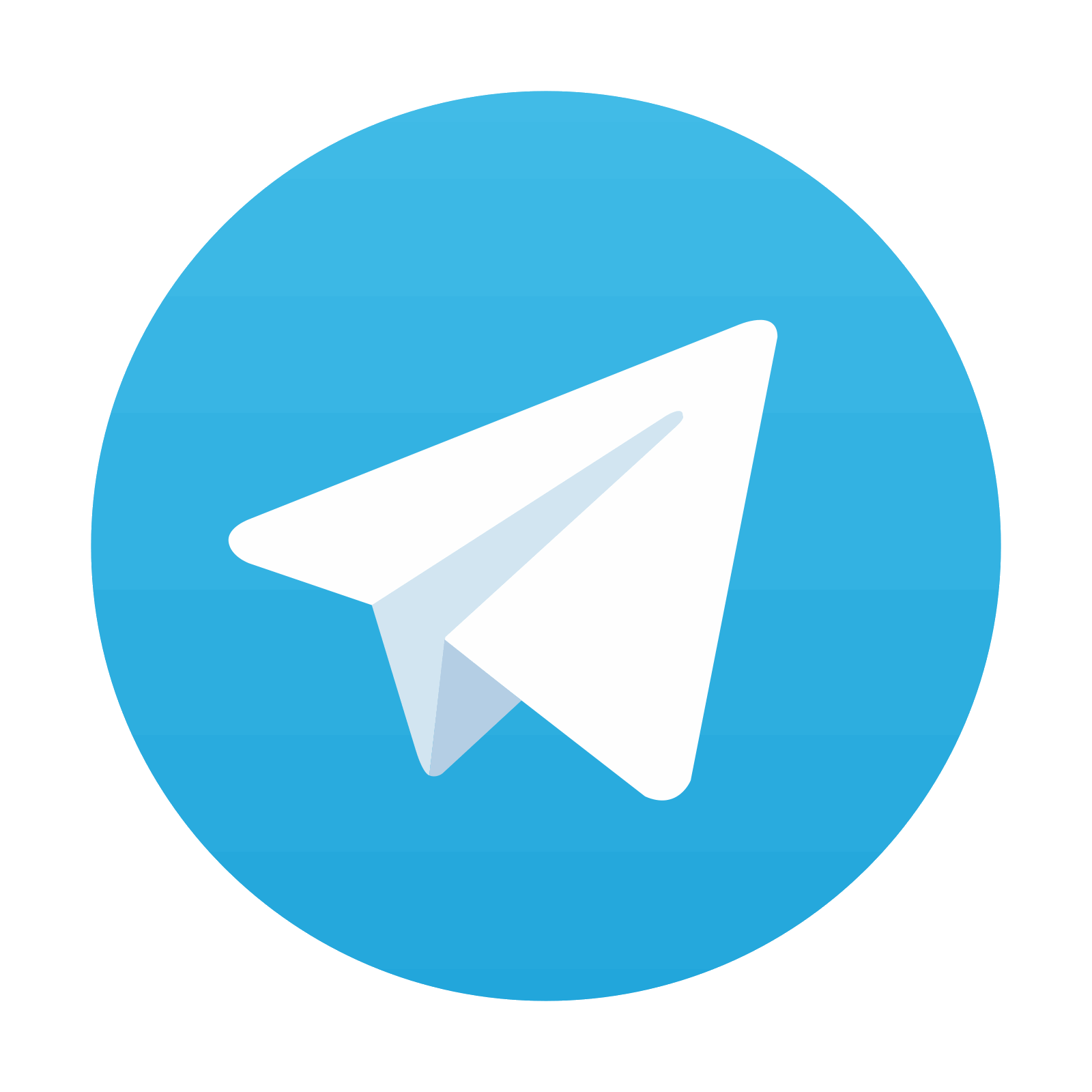
Stay updated, free articles. Join our Telegram channel
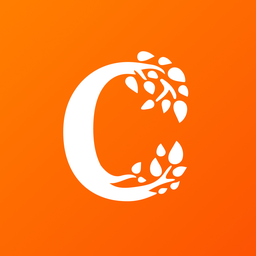
Full access? Get Clinical Tree
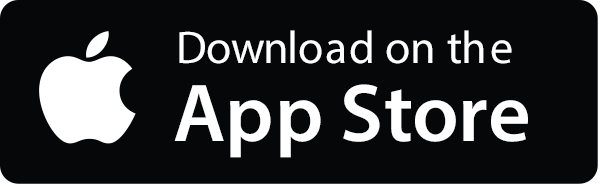
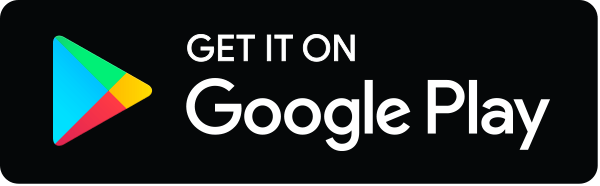
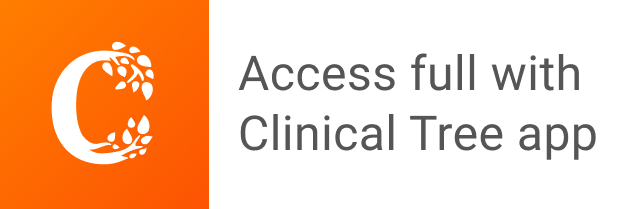