Fig. 16.1
Normal lumbar spine
16.1.1 Vertebrae
The five lumbar vertebrae are aligned with a prominent convex curvature in the lateral or mid-sagittal plane, otherwise known as lordosis. Lumbar vertebrae consist of an outer casing of dense and compact cortical bone. The interior part of the body is composed of cancellous bone aligned in lattice manner to resist axial force while minimizing mass, the effectiveness of which is proportional to its density. Lumbar vertebrae consist of the large body ventrally and posterior elements dorsally (Fig. 16.1). Extending from the vertebral body and moving posteriorly are the pedicles, transverse processes, articular processes forming the facet joints, laminae, and spinous processes. The vertebral foramen is a prominent feature of the lumbar vertebrae and is formed by the posterior aspect of the vertebral body and medial aspects of the pedicles, articular processes, and laminae. Extended from the base of the cranium and formed by all 33 vertebral foramina is the spinal canal, through which the spinal cord traverses. The cord transforms into the cauda equina near the second lumbar vertebra. Nerve roots exit the spinal canal at every vertebral level through the intervertebral foramina, formed by the postero-lateral aspect of the vertebral body, cranial and caudal aspects of opposing pedicles, and the anterior aspect of the articular processes.
The vertebral bodies form the most massive portion of the vertebra, are located anteriorly, and are largest in the lumbar region, compared to thoracic and cervical regions. Lumbar vertebral bodies have relatively flat and kidney-shaped cranial and caudal surfaces. Transverse processes extend laterally from the pedicles. Facet joints form between opposing surfaces of the articular processes with joint surfaces oriented ventral-laterlly for the cranial processes and dorso-medially for the caudal processes. The pars interarticularis lies between superior and inferior facet joint processes. The large, flat, and vertically oriented spinous processes are located at the dorsal aspect of the vertebrae, are largest in the cranial region and decrease in size at the caudal levels. The five bony vertebrae of the lumbar spine are interconnected by soft tissues and joints, as described below.
16.1.2 Endplates
The endplates are the cranial and caudal surfaces of the vertebral bodies and form a thin cartilaginous interface between the bony vertebral body and the fibrocartilaginous intervertebral disc. The endplates are composed of hyaline cartilage. They are fused to the vertebral body by a calcium layer through which small pores penetrate for the nutrition of the intervertebral disc. The inferior zone of the vertebra remains in contact with the cartilage by the lamina cribrosa, the sieve-like surface. Osmotic diffusion occurs through this layer.
16.1.3 Intervertebral Discs
Intervertebral joints consist of a form of cartilaginous joint known as a symphysis. The primary component of the symphysis is the intervertebral disc, although the endplate (discussed above) is also a component. Lumbar intervertebral discs are located between adjacent vertebral bodies from the thoracolumbar junction (T12–L1) through the lumbo-sacral junction (L5–S1) and are connected to the bodies by the endplates. Their concentrically arranged components are: the outer alternating layer of collagen fibers forming the peripheral rim of the annulus fibrosus; a fibrocartilage component forming a major portion of the annulus fibrosus; the transitional region between the central nucleus pulposus (core), where the annulus fibrosus and the nucleus pulposus merge; and the nucleus pulposus made of a soft, pulpy, highly elastic mucoprotein gel containing various mucopolysaccharides, collagen matrix and water with relatively low collagen fibril. Intervertebral discs attach to the vertebral bodies centrally via the endplates and peripherally via the annulus fibers and ligaments. While some of the annulus fibers blend into the anterior and posterior longitudinal ligaments, others attach to the rim of the vertebra. The disc resists axial compression and tension, lateral and antero-posterior shear, and axial rotation. The shape of the disc in the lumbar spine is such that the lordotic curvature is maintained by the greater height ventrally than dorsally.
16.1.4 Ligaments
Ligaments are multilayered and composed primarily of elastin and collagen in different rations. In very general terms, collagen adds strength to the ligament whereas elastin adds elasticity. Spinal ligaments connect adjacent vertebrae (e.g., interspinous ligament) or extend over several segments (e.g., anterior longitudinal ligament). They are uniaxial structures. As such, they are capable of resisting only tension and buckle under compression. The anterior longitudinal ligament (ALL) runs from the occiput to the sacrum. It consists primarily of long, arranged collagen fibers which are aligned in interdigitizing layers. The deep layer extends only to adjacent vertebrae, the middle layer over a few vertebral levels, and the outer over four to five levels. This stratification is of significance in regulating physiologic motion. This ligament functions to prevent hyperextension and excessive distraction; it is functionally active in extension and rotation. The posterior longitudinal ligament (PLL) originates from C2, continues to the coccyx, and is located on the dorsal surface of the vertebral bodies. While this multilayered ligament closely adheres to the disc annulus, attachments to the vertebral bodies are minimal. It is broader in the area of the intervertebral disc and very thin in the area of the vertebral bodies. Deeper layers span only the intervertebral disc and superficial layers can extent across multiple vertebral segments. The cross-section is considerably smaller and the tensile response is weaker than ALL. The ligamentum flava span between adjacent surfaces of laminae and are discontinuous, spanning from the cranial surface of the caudal vertebra to the caudal surface of the cranial vertebra. Ligamentum flava are present from C2–C3 to the sacrum. These ligaments are also termed yellow ligaments due to their appearance. Laterally, it is confluent with the joint capsules. Eighty percent elastin, and under some tension preload at rest, ligamentum flava are quite effective in returning the laminae to their resting positions following flexion. Capsular ligaments are intimate to the facet joint capsule and attach to the vertebrae adjacent to the articular joint. Their fibers are aligned normal to the facets, limiting distraction and sliding of the facet joints and hyperflexion of the segment. The interspinous ligaments connect adjacent spinous processes and are met by the ligamentum flavum anteriorly and the supraspinous ligament posteriorly. The supraspinous ligament is a fibrous ligament running along the distal extent of the spinous processes from seventh cervical vertebra to the sacrum. Interspinous and supraspinous ligaments act to resist flexion bending.
16.1.5 Facet Joints
Facet joints are articular joints that are located postero-laterally to the intervertebral disc at each vertebral segment (T12–L1 through L5–S1). There are two facet joints per segment (right and left sides). The joints are formed by the superior articular process from the caudal vertebra (facing dorso-medially) and the inferior articular process from the cranial vertebra (facing ventro-laterally). Opposing surfaces of the articular processes consist of a smooth and resilient layer of hyaline or articular cartilage. Surrounding the joint is a joint capsule and capsular ligament. Capsular ligaments are composed primarily of collagenous fibers and provide resistance to joint distraction that can occur during a variety of segmental movements. The joint capsule, also known as the synovial membrane or articular capsule, forms a complete envelope around the joint and acts to maintain joint integrity by containing the synovial fluid. The synovial fluid facilitates articulations and allows for ‘gliding’ of opposing articular surfaces that occurs during physiologic motions including flexion/extension, lateral bending, and axial rotations.
16.2 Injuries to the Spine
Injuries to the lumbar spine result from direct violence to the column or specific vertebrae. Unlike penetrating trauma, violence to the spine can most commonly be attributed to gross motions or acceleration of the body/torso. Examples include anterior bending of the torso resulting in flexion loads on the lumbar spine or vertical acceleration of the pelvis leading to axial compressive loads on the lumbar column. Loads placed on the tissues lead to deformation, with the magnitude, rate, and direction/type of loading responsible for the tissue distortion profile. Injury occurs when deformation exceeds physiologic limits of the tissue. The type and location of tissue deformation is dependent upon the applied load. Pure loads can take the form of linear forces or rotational bending moments. Linear forces can be applied in any direction, but are generally broken down into components based on axial tension/compression perpendicular to the horizontal plane, anterior-posterior shear perpendicular to the frontal plane, or lateral shear perpendicular to the sagittal plane. Likewise, bending moment components include flexion/extension in the sagittal plane, lateral flexion in the coronal plane, and axial twist in the horizontal plane.
16.2.1 Injury Classification
Lumbar spine injuries can be classified according to loading mechanism. Injuries can occur under tension, compression, shear, or bending, although some of these mechanisms are less common due to the inherent characteristics of the in situ lumbar spine. Compression-related injuries are most common type in the thoraco-lumbar spine [1, 2]. Compression injuries occur in any number of high-rate axial loading scenarios including parachuting, falls from height, and motor vehicle crashes [3–8]. These injuries also occur in a variety of military- and sporting-related activities including skiing, snowboarding, and other sporting accidents [9–12], aviator ejection [13–20], helicopter crash [21–23], and underbody blast [24–31]. Injury mechanisms involve the primary component of axial force that can be coupled with varying magnitudes of bending. Injury type and severity are controlled by the biomechanical aspects of the insult. Specifically, axial force applied through the center of rotation of an intervertebral segment results in burst fracture. Axial forces offset from the center of rotation of a segment develop coupled bending moments, with greater offset distances resulting in greater moment magnitudes. Axial forces offset anteriorly, posteriorly, or laterally result in flexion-related injuries, extension-related injuries, or lateral bending-related fractures, respectively.
Tension and shear injuries can also occur in the lumbar spine. Tension (distraction) of the lumbar column is not a common loading scenario for humans. However, localized distraction occurs in different tissues during bending. For example, during forward flexion the anterior structures of the lumbar spine sustain localized compression loading. However, tissues posterior to the axis of rotation are distracted. This is particularly relevant for soft tissues such as ligaments and facet joint components, although bony fractures can also result from localized distraction (see Chance Fracture below). Likewise, shear injuries can affect he lumbar spine in some cases. However, the coupled effect of abdominal tissues most often transforms shear loading applied to the abdomen into bending of the spine. This section outlines different types of lumbar spine fractures, highlighting biomechanical mechanisms and briefly indicating the acute clinical outcome.
Burst fractures result from pure compression transmitted directly along the line of the vertebral bodies (Fig. 16.2). Due to the inherent lordotic curvature of the lumbar spine, pre-flexion is necessary to induce a purely compressive state [32]. From a biomechanical perspective, a relatively uniform compressive load is applied across the axial plane of the vertebral body. This results in loss of vertebral body height due to fracture of anterior and posterior cortices. Axial force also leads to fracture of one or both endplates, forcing the intervertebral disc nucleus into the vertebral body, and resulting in a burst pattern [32, 33]. High energy fractures can result in retropulsion of bony fragments into the spinal canal and associated neurological deficit. Posterior element fractures may also be involved, but are not required for this classification. Ligaments commonly remain intact and the spine is mechanically stable. These injuries have been considered to be clinically stable or unstable. Ferguson and Allen reported that these fractures were generally stable, but other clinicians have reported progressive neurological injury or advancing post-injury deformity [33–35].
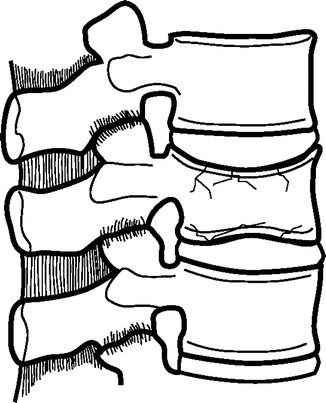
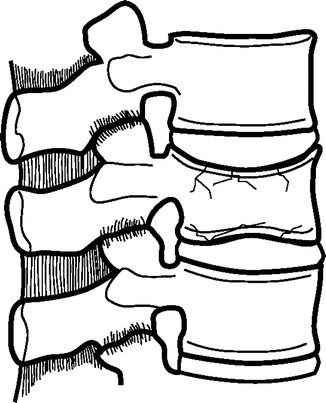
Fig. 16.2
Burst fracture
Anterior wedge fractures result from axial compression combined with flexion [36] or flexion alone [32] (Fig. 16.3). This combination can result from axial loads applied anterior to the center of rotation of the vertebral segment or axial loading combined with anterior bending of the torso. In terms of biomechanics, tissues anterior to the center of rotation (e.g., anterior aspect of the vertebral body) sustain compression whereas middle- and posterior-column tissues (e.g., dorsal to the sagittal plane center of the vertebral body) are subjected to tension. Fractures affect the vertebral body and involve greater loss of body height anteriorly than posteriorly. In many cases, the posterior body height is unaffected. This results in a wedge-shaped profile as seen on lateral X-rays. While dynamic compressions are likely greater, wedge fractures typically present with less than 50 % anterior body height loss due to post-injury restitution. Slight and moderate wedge fractures are stable as the posterior aspect of the vertebral body and posterior ligamentous complex remain primarily intact [37]. Severe wedge fractures can occur with or without disc injury and involve disruption of the posterior ligamentous complex including ligamentous rupture or spinous process fracture. These fractures are unstable and typically referred to as fracture dislocations, which will be discussed below.
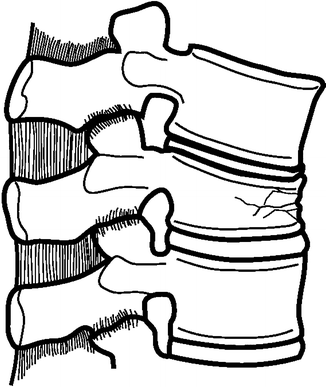
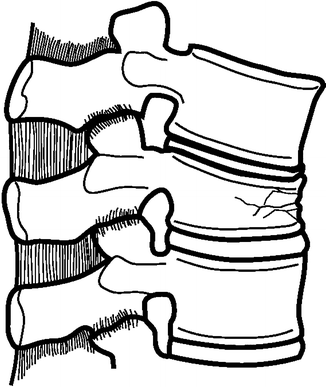
Fig. 16.3
Anterior wedge fracture
Wedge fractures can also occur laterally. Two mechanisms have been proposed to result in lateral wedge fractures. Flexion combined with rotation is the more commonly cited mechanism [2, 36], although Ferguson implicated compression combined with lateral bending [33]. Both mechanisms result in unilateral wedging, with the opposite side remaining intact, attributed to compression on the concave side and tension on the convex side. Radiographically, these injuries are evident in a frontal plane wedge-shaped profile when viewed on anterior-posterior X-rays. The lumbar column may also appear to have a lateral curvature centered about the injured level. Nicoll describes a unilateral wedging combined with transverse process fracture on the convex side and posterior intervertebral joint fracture on the concave side [2]. These injuries are generally considered to be clinically unstable, often associated with prolonged unilateral neurological deficit.
Fracture dislocation is a generic terms that refers to a condition involving fracture of the vertebra coupled with dislocation [36] (Fig. 16.4). Dislocations can also occur in the absence of any bony fracture. These injuries commonly include rupture of the posterior interspinous ligament [2]. Depending on the status of the capsular ligaments, facet dislocation can occur, resulting in conditions involving upward subluxation, perching, forward dislocation, or forward dislocation with locking [2]. Kaufer and Hayes classified fracture dislocations into five groups based on the presence and type of anterior and posterior fracture or dislocation [38]. The mechanism for these injuries often involves flexion coupled with axial rotation or lateral bending. The coupled bending component is necessary in the lumbar spine region due to the inherent stability of the column, which can be attributed to the large vertebral bodies, wide and flat intervertebral discs, and well developed longitudinal ligaments. In the case of axial rotation, one or both articular processes can fracture causing the upper vertebra to rotate about the lower, breaking off a wedge-shaped section from the anterior region of the inferior vertebral body. A large coupled shear component can also contribute to fracture dislocations [36]. Fracture dislocations are clinically unstable with a propensity toward progressive deformity and acute neurological deterioration [2, 33, 38].
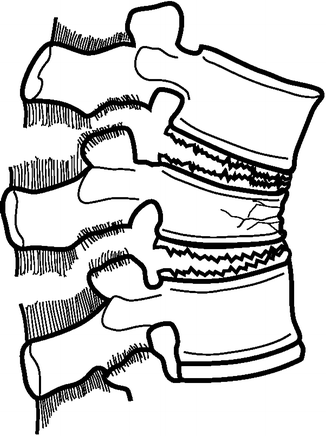
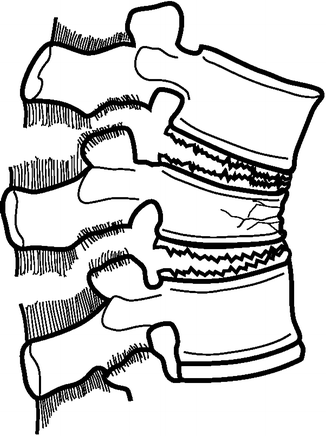
Fig. 16.4
Fracture dislocation including body fracture and facet dislocation
Chance fractures were first described by G.Q. Chance in 1948 [39] (Fig. 16.5). The mechanism of injury involves flexion coupled with distraction. Fractures initiate in the posterior aspect of the neural arch, often including the spinous process, and extend anteriorly into the posterior aspect of the vertebral body, terminating in an upward curve that extends toward the superior endplate just anterior to the neural foramen. These fractures occur in the absence of anterior vertebral body disruption or dislocation of the facet joints. The mechanism of these injuries is attributed to hyperflexion plus distraction. In many cases, these injuries were thought to result from the use of a lap seatbelt in automobile collisions [40, 41]. This mechanism is particularly relevant for improperly positioned lapbelts or pediatric occupants with immature pelvis to support the restraint [40–42], although other authors claim these injuries are rare in children 43. In these cases, the lapbelt would function as a fulcrum for the spine to rotate about, resulting in tension injuries of the posterior lumbar vertebra. Incorporation of a properly worn shoulder belt to support the torso has minimized the likelihood of these injuries. From a clinical perspective, these injuries are considered to be stable, with little chance of neurological deficit [2, 42].
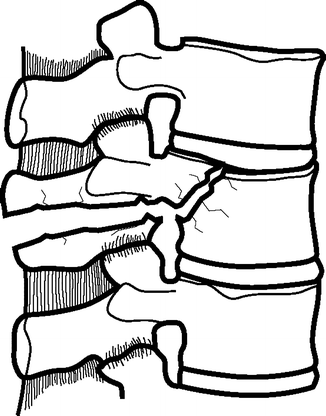
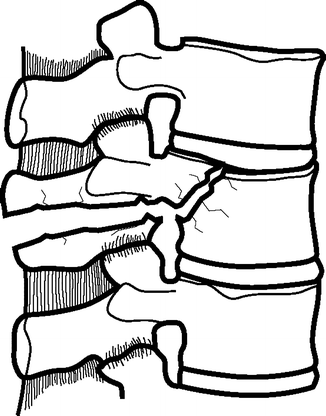
Fig. 16.5
Chance fracture
16.3 Loading Issues
Numerous biomechanical investigations of the lumbar spine have been conducted using whole body cadaveric specimens, lumbar columns, spine segments, and isolated tissues. These investigations have quantified quasi-static and dynamic physiologic, degenerated, and traumatic responses of the lumbar spine under a variety of loading conditions including compression, bending, and shear. A comprehensive review of lumbar spine biomechanical research is beyond the scope of this chapter. Rather, this chapter aims to highlight experimental biomechanical methods and provide tolerance-related information for the lumbar spine. Due to the prominence of axial loading on lumbar spine injury mechanics, a significant portion of existing research has focused on this mode. Accordingly, this chapter will focus primarily on experimental studies of axial loading. The following sections provide a description of three experimental protocols that have been used to investigate dynamic axial tolerance of lumbar spine components, and a fourth method incorporating whole body specimens.
16.3.1 Electro-hydraulic Testing Device
A considerable amount of experimental effort has been applied toward the understanding of lumbar spine injury tolerance during dynamic axial loading. Much of that research has been conducted using either electro-hydraulic testing devices or weight-drop apparatuses. Research incorporating electro-hydraulic testing devices have been conducted using whole lumbar columns [44–46], column segments (e.g., two-vertebra motion segments) [47–49], isolated vertebral bodies [50–58], and components including ligaments and annular tissues [59–75]. These devices apply quasi-static or dynamic axial loads using the piston of the electro-hydraulic device. An advantage of electro-hydraulic testing devices is that piston excursion is computer controlled, which leads to a high level of control over the loading versus time pulse, although these devices are somewhat limited in loading rate. Loads can be distributed across the vertebral endplate, applied at a distance from the specimen to induce a bending moment, or locally applied to a specific region of the endplate or intervertebral disc using an indentor. By design, electrohydraulic testing devices are typically uniaxial and compression is the most common loading mode. However, the devices can also impart compression combined with bending through application of axial load at a distance from the center of segmental or column rotation using a moment arm or vertebral arch (Fig. 16.6). For example, compression-flexion loading can be induced through load application to a moment arm extending anteriorly from the cranial vertebra. Likewise, compression-extension and compression-lateral bending are induced using moment arms extending posteriorly or laterally. Oblique loading can be produced through application of the load in the anterior- or posterior-lateral locations. Distance of the load application from the center of rotation controls the ratio of axial load to bending. Load application at a greater distance from the center of rotation results in a higher ratio of bending to axial load. Likewise, load application closer to the center of the vertebra leads to a higher component of compression.
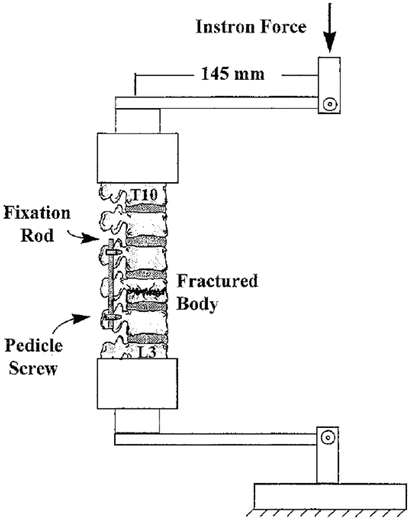
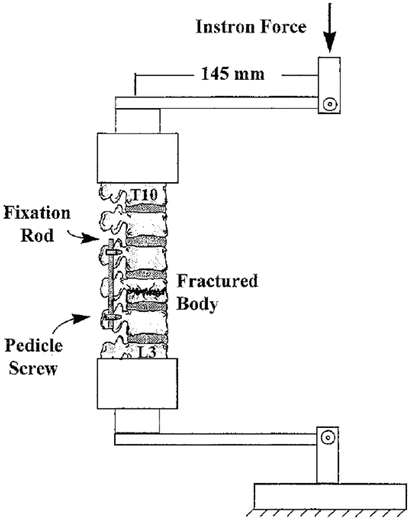
Fig. 16.6
Experimental model incorporating an electro-hydraulic testing device to induce compression-flexion on a lumbar spine column (From Mermelstein et al., Spine 1998 with permission)
Testing is generally conducted with specimens in neutral position, although some series have applied flexion or extension loads to bias the initial position and study orientation effects [48]. Custom devices have the ability to apply axial tensile or compressive forces at loading rates up to 9 m/s. However, testing of lumbar columns and segments has generally been conducted at quasi-static rates as low as 1.0 mm/min [47] or dynamic rates up to 1.0 m/s [46]. Testing of individual vertebrae has been conducted at rates up to 2.5 m/s [51]. Specimens can be instrumented, with reflective markers, accelerometers, and strain gauges to obtain level-by-level kinematic (displacement and angulation) or localized compressive information. Loads at the impacted and distal ends are recorded using load cells and localized (level-specific) loads can be computed by coupling load cell data with kinematics information. Because of the controlled nature of load application using the piston, dynamic subfailure loading can be used to quantify the physiologic response of lumbar tissues. Likewise, injuries produced during dynamic loading can be correlated with biomechanical measures to derive injury tolerance information. However, it is difficult to achieve a constant velocity during biofidelity testing as the piston has to initiate its travel from rest, and deceleration initiates prior to the point of peak displacement. Constant loading rates can be obtained with piston overshoot, by setting piston displacement to a maximum level well beyond the expected fracture displacement. Inertial effects of the piston require compensation for force measurements from load cells attached to the piston and in-line with the loading vector.
Electro-hydraulic testing devices are also useful for testing of isolated tissues under appropriate loading modes. Although tension of the lumbar spine is rare, specific soft tissues sustain tension during different loading situations. For example, dorsal soft tissues sustain tension during segmental flexion. Likewise, due to Poisson’s effect, intervertebral disc annular tissues sustain tension during segmental compression. Similar to compression, tension loading rates can vary from quasi-static to dynamic and maximum distraction can be maintained within the physiologic range or can enter the traumatic realm. A variety of biomechanical studies have been conducted using electro-hydraulic testing devices to quantify tensile response of lumbar spine ligaments [63–65, 67, 68, 70, 72] and intervertebral disc material [60, 61, 66, 76].
16.3.2 Weight-Drop Apparatus
The weight-drop apparatus is another method of load application similar to the electro-hydraulic testing device, with the primary exception that loads are applied by dropping a weight onto the cranial end of the specimen instead of using the piston (Fig. 16.7). Numerous studies have been conducted using the weight-drop apparatus and incorporating 2- and 3-vertebrae segments [77–79] or longer lumbar columns [80–83]. This test setup applies dynamic compressive loads by impacting the spine using a decelerated weight. The weight is accelerated by gravity until impacting the spine and is either guided or allowed to fall without constraint. Similar to the electro-hydraulic testing device, compression is the most common loading mode, although compression combined with bending can be applied through application of axial load at a distance from the center of segmental or column rotation using a moment arm.
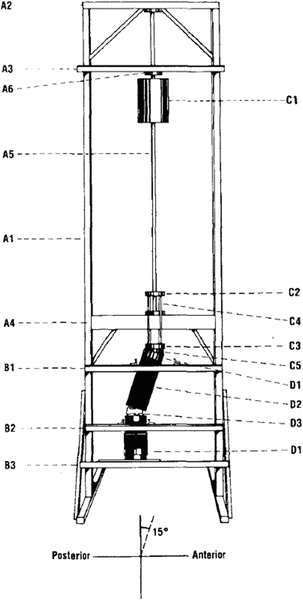
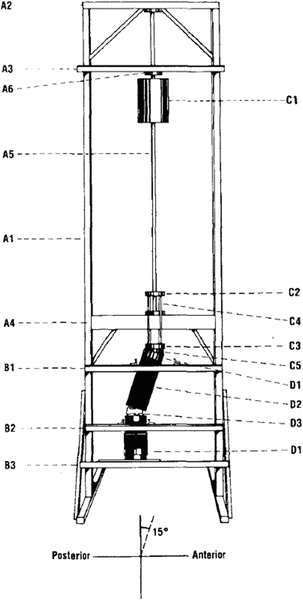
Fig. 16.7
Experimental model incorporating a weight-drop device to induce compression-flexion on a lumbar spine column (From Cotterill et al., J Orthop Res 1987 with permission)
Testing is most commonly conducted with specimens in neutral position, although pre-flexion has been applied in some cases [81, 84, 85]. Rate of loading is controlled by mass of the impactor and its closing velocity (i.e., velocity at the time of impact). Control of maximum compression is difficult as this method relies on specimen impact to halt the excursion of the dropped weight. Loading rate can be quantified as the rate of load application (N/s). Specimens can be instrumented, with reflective markers, accelerometers, and strain gauges to obtain level-by-level kinematic (displacement and angulation) or localized compressive information. Loads at the impacted and distal ends are recorded using load cells and localized (level-specific) loads can be computed by coupling load cell data with kinematics information. Subfailure testing is performed by dropping the weight from a height that does not induce fracture and is used to quantify the physiologic response of lumbar tissues. Likewise, injuries produced during dynamic loading can be correlated with biomechanical information to derive tolerance information. However, it is difficult to achieve a constant velocity during biofidelity testing as deceleration of the dropped weight initiates immediately upon contact with the specimen.
16.3.3 Drop Tests
Drop tests are used to replicate vertical acceleration conditions in either component or whole body cadaver tests (Fig. 16.8). Benefits of this experimental setup realistic loading and boundary conditions and the ability to relate injury tolerance to external metrics associated with the loading environment (i.e., acceleration of the lumbar spine base). These tests involve a drop tower of varying height, at least one platform connected to a guide rail using linear bearings or a cart mechanism, and pulse-shaping material at the bottom of the tower to modulate characteristics of the deceleration pulse. Testing involves mounting the specimen to the platform, raising the platform to a specific height, release with gravity accelerating the platform downward, and impact to the pulse-shaping material at the base of the drop tower. Characteristics of the deceleration versus time pulse are controlled using initial height, mechanical properties of the pulse-shaping material, and amount of pulse shaping material. Peak accelerations as high as 65 G with rates of onset as high as 2,500 G/s have been achieved using this model [86]. In general, greater peak accelerations can be obtained with drops from greater initial height and steeper rates of onset (i.e., shorter pulses) are obtained with stiffer pulse-shaping material. In the case of isolated components, similar biomechanical information can be collected to that for the weight-drop and electro-hydraulic testing devices including forces at the top and base of the specimen, three-dimensional spinal kinematics (e.g., linear and angular motions and accelerations), localized strains, and fracture information from acoustic sensors. A second decoupled platform with variable mass can be added to the cranial aspect of the spine to simulate the torso. The mass can be the same for all specimens tested under a given protocol, for consistency, or can be varied to mimic specimen-specific torso mass. Lumbar spine components can be tested in compression or, since the mass can be attached to the upper platform via a telescoping linkage and load application can be moved anterior-posteriorly, compression combined with flexion, extension, or lateral bending.
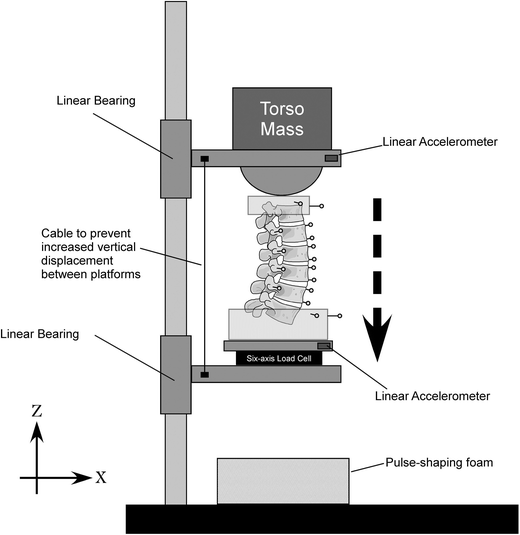
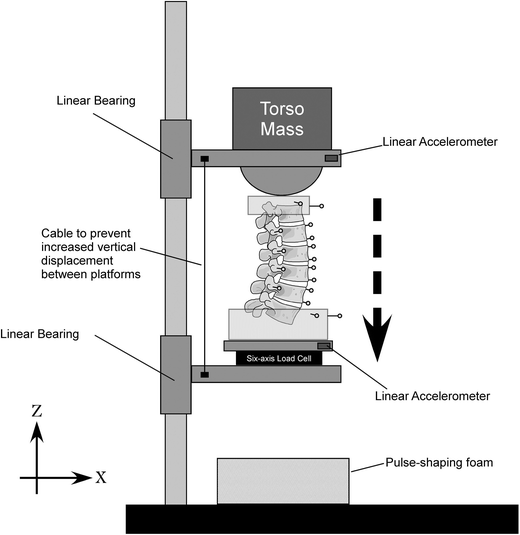
Fig. 16.8
Experimental model incorporating a drop tower apparatus to induce compression-flexion on a lumbar spine column (From Stemper et al., J Biomech Eng 2011 with permission)
16.4 Specimen Details
Different types of experimental models exist to determine the biomechanical properties, replicate real-world injuries, derive injury mechanisms, and determine human tolerance in terms of variables such as forces and risk curves using the above described experimental techniques. Some of the more common experimental models are discussed in this section.
16.4.1 Isolated Components
Testing of isolated components such as vertebral bodies has been performed to quantify the structural or material response of the isolated vertebral body or endplate. Testing of vertebral bodies and endplates is typically performed using electro-hydraulic testing device with flat horizontal plate for bodies and an indentor for endplates [51, 87–90]. These tests have been conducted from quasi-static to dynamic rates. Axial force is measured using a load cell, compression displacement is measured using two-dimensional videography or the piston LVDT, and vertebral body strain is measured using strain gauges. These types of testing are ideal for the quantification of high-rate material properties as loading conditions are controlled, repeatable, and applied directly to the tissue of interest.
Isolated soft tissues have also been tested to quantify the structural and material response. Those tests are typically conducted by distracting the specimen in tension using an electro-hydraulic testing device [61, 70]. Testing has quantified the quasi-static, dynamic, and viscoelastic response of isolated ligaments and annular tissues. Test specimens are commonly arranged in an I-shaped mechanical test specimen. However, attachment to the test frame can be difficult for smaller tissues (i.e., fascicles) and test coupons may be required [64].
16.4.2 Segmented Columns
Segmented column models are used to experimentally delineate the gross biomechanical responses of the spine at a macro level and determine tolerance characteristics. Effects of lordotic curvature are incorporated because more than one functional unit is used. Pre-alignment of the spine can be incorporated to account for effects of body posture. However, the degree of inclusion of these factors depends on the number of spinal segments. Consequently, these models tend to be more realistic from injury reproduction perspectives although failure responses of individual components cannot be quantified because the load-path at a segmental level is unknown. Three-dimensional motions of the intervertebral levels have been obtained at high rates of 1,000 samples per second. Two-dimensional motions using high resolution digital cameras can be obtained at much higher rates (~50,000 frames per second). Likewise, local accelerations and strains of individual vertebrae can obtained using accelerometers, strain gauges, and acoustic emission sensors to determine the timing of fracture or spinal instability. Positioning the segmented column on an x–y cross table mounted to the platform of an electro-hydraulic testing device is needed to achieve the intended posture or pre-alignment. The transmitted forces and moments can be recorded at the inferior end using a six-axis load cell. Forces and moments at the segmental level of injury may be estimated although the local dynamics are not known. High-speed video images can be taken to document macroscopic failures, high-speed x-rays can be obtained for bony fractures, and localized segmental motions analyses can be performed using this model. Strict control of the experimental loading conditions can be achieved using segmented columns. Testing can be conducted at injurious levels, or below the threshold for injury to quantify the physiologic response.
Another methodology to apply dynamic loads to the segmented column is using free-fall or drop techniques as described above. This involves fixing the ends of the column, applying preloads (if any), controlling alignment by techniques such as pre-flexing using cables, and dropping on to targets with known stiffness to modulate the pulse. Ensuing motions of the column following initial contact with the target may induce continuing loads and contribute to additional injuries. However, load limiters have been used to prevent this occurrence.
16.5 Biomechanical Data
A number of studies have been performed to characterize lumbar spine fractures and quantify biomechanical tolerance due to axial loading. Specific aims of these studies were to clarify the injury mechanism, observe fracture patterns, measure spinal canal occlusion, compare surgical instrumentation techniques, or understand biomechanics of injury. As mentioned above, in many cases, the electro-hydraulic testing device or weight drop models were employed. However, other studies have incorporated the alternative models described above. Physiologic and injury tolerance information has been derived from these studies. Although not comprehensive, some of the relevant findings are discussed below.
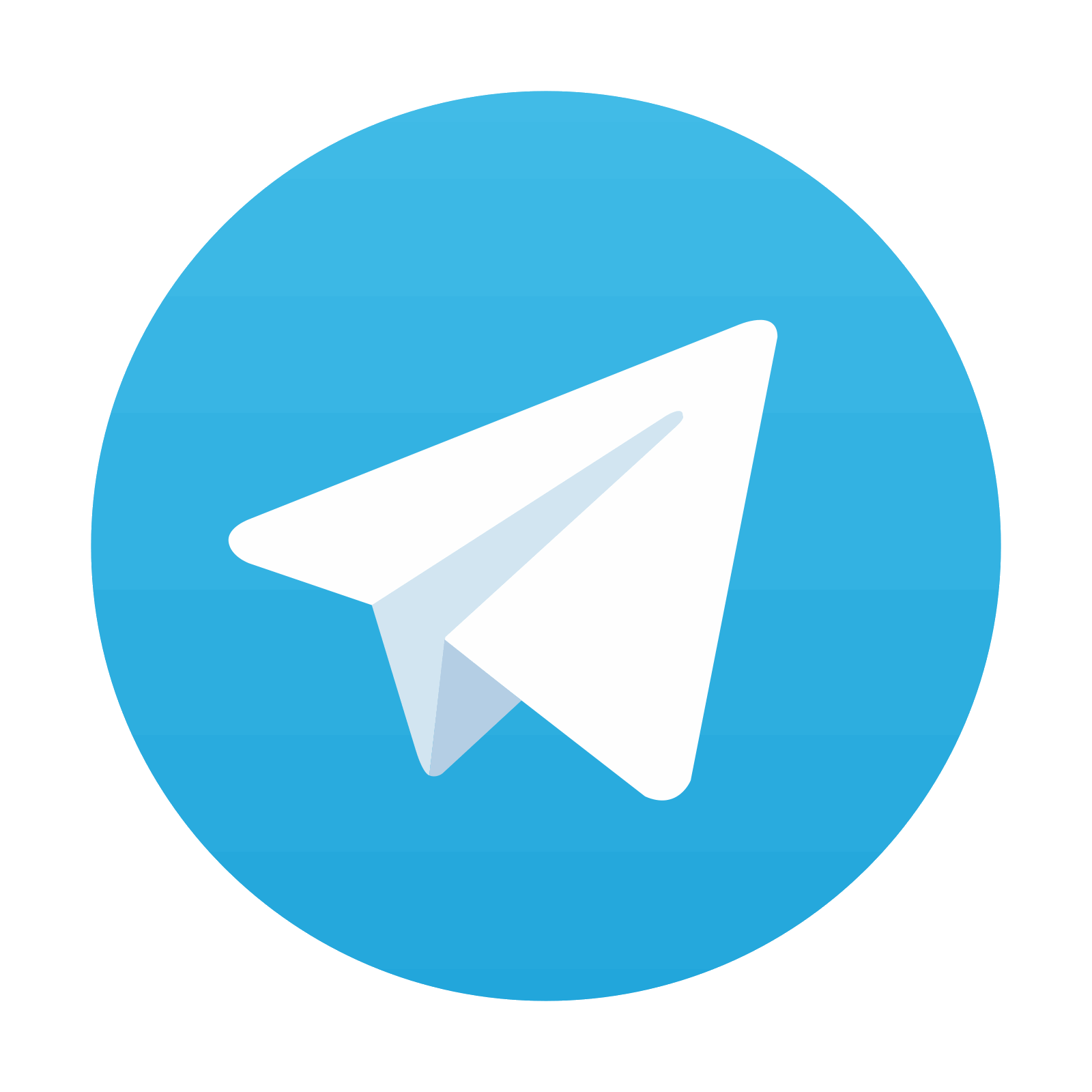
Stay updated, free articles. Join our Telegram channel
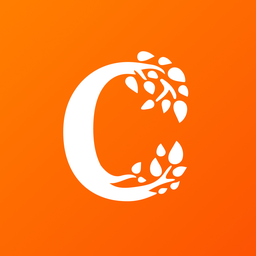
Full access? Get Clinical Tree
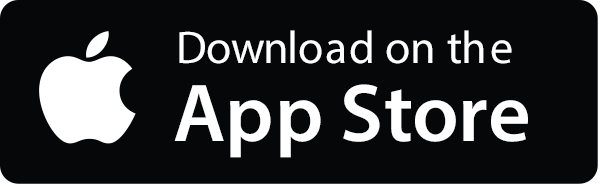
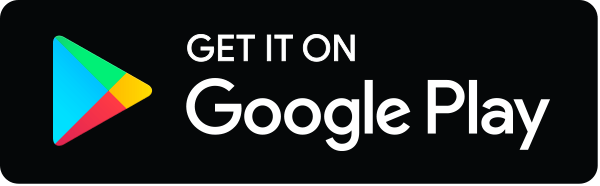