Fig. 3.1
(Upper): oblique diagram of an intervertebral disk. The nucleus behaves like a pressurised fluid and is restrained by tensile stresses (T) in the surrounding annulus. Compressive loading (C) is evenly distributed across most of the disk. (Lower): expanded detail of annulus, showing the alternating collagen fibre direction (α) in adjacent lamellae. Typically, α = 30°. Also shown are the number of lamellae (N) and the number of collagen fibre bundles that cross a typical vertical section (n) [116]. Adapted from Adams et al. [15] with permission
The disk nucleus contains a high concentration of proteoglycans, which attract water into the tissue and ensure that it behaves like a pressurised fluid, distributing load evenly on the adjacent vertebral bodies. The annulus is mostly comprised of coarse collagen type I fibres which run obliquely between the vertebrae (Fig. 3.1). Annulus tissue is sufficiently soft to allow small intervertebral movements and yet strong enough to prevent excessive movements and to restrain the nucleus. Disks are too stiff to act as efficient shock absorbers.
3.1.2 Disk Injury or Degeneration?
Disks must distribute forces evenly on the vertebral bodies, even when the spine is heavily loaded in flexed or extended postures. This is a physically demanding role, especially in the lower lumbar spine of humans, and disks often show signs of structural damage even by the age of 20 years [1]. The traditional orthopaedic view of intervertebral disk pathology emphasised structural lesions [2] and physical causes [3, 4]. More recently, however, this common-sense approach has been overshadowed by the ‘disk degeneration’ paradigm, which considers that some biological process related to genetics and disk nutrition can be held responsible for all aspects of disk pathology and pain. Mechanisms of disk injury, either by wear and tear (‘fatigue’) or by trauma, have been overlooked on the grounds that they represent an outmoded ‘injury model’, with some advocates of degeneration appearing to deny the possibility that intervertebral disks can be injured at all!
And yet, there is increasing evidence that intervertebral disks often are injured and that injury leads directly to altered biology, including nerve ingrowth and pain. Animal experiments show that disk injury leads inevitably to degeneration [5–7], and longitudinal studies on humans confirm that disk degeneration usually follows an injury to a disk or vertebral body [8, 9]. Epidemiological studies show that excessive mechanical loading is associated with disk degeneration and prolapse [10–12], although we now suspect that moderate mechanical loading actually strengthens spinal tissues by the process of adaptive remodelling [13, 14]. All of the structural features of disk degeneration (such as annulus fissures, nucleus herniation, endplate defects and internal disk disruption) can be reproduced by excessive mechanical loading applied to cadaveric spines, and the failure mechanisms have been explained by mathematical models [15]. No ‘disk degeneration gene’ has been found, only a range of gene variants (alleles) which appear to exert their influence by weakening the extracellular matrix of the disk [16, 17] or bony endplates [18]. Finally, we now know that metabolite transport into the disk increases with advancing age and degeneration, as the vertebral endplates become more porous and permeable [19], suggesting that inadequate disk nutrition is unlikely to be a direct cause of disk degeneration.
3.1.3 Purpose and Scope of This Chapter
The purpose of this chapter is not to revive some old ‘injury’ model of intervertebral disk failure, but to integrate old and new evidence concerning disk injury with that concerning disk biology, pathology and pain. Essentially, the following sections will show how some intervertebral disks can be so weakened by genetic inheritance and by advancing age that they fail mechanically in response to specific types of everyday mechanical loading. Attempts at healing are frustrated by low cell density and the harsh mechanical environment, so that disk cell metabolism becomes increasingly abnormal (‘degeneration’) and structural disruption increases. Eventually, blood vessels and nerves are able to grow into the disrupted tissue, and the disk becomes painful.
The chapter begins with an account of forces acting on the spine, because many clinicians consider only gravitational forces and overlook the much greater forces that arise from muscle tension and from accelerations. This is followed by a detailed account of how excessive forces can cause specific types of structural damage to lumbar disks and their adjacent vertebrae, including disk herniation. Damage can be caused by injury or by fatigue (‘wear and tear’) loading, and forces need not be high if the tissues are weak. Subsequent sections compare disk ageing and degeneration, because they are not the same thing: ageing is only one of several risk factors for degeneration, which is not inevitable, not even in old age.
3.2 Forces Acting on the Lumbar Spine
3.2.1 Compression, Shear, Bending and Torsion
The nature of these forces can be appreciated from Fig. 3.2a. The compressive force denotes the force acting down the long axis of the spine, perpendicular to the mid-plane of each intervertebral disk. Note that its direction varies with spinal level and with posture. Compression is resisted mostly by the intervertebral disks and vertebral bodies [20]. The shear force acts parallel to the mid-plane of each disk and is steeply inclined to the horizontal in the lower lumbar spine. Forward shear is resisted by the articular surfaces of the apophyseal joints as well as by the disk [21]. A ‘bending moment’ (force X distance) causes the spine to bend forwards, backwards or laterally. Forward and backward bending are resisted primarily by spinal ligaments [22] and the neural arch [23], respectively. Torsion (axial rotation) twists the spine about its long axis and in the lumbar spine is resisted primarily by the neural arch [24].


Fig. 3.2
(a) Human lumbar spine showing the direction of the spinal compressive force (C) and shear force (S) acting on the L5–S1 disk. A bending moment (BM) causes the spine to flex, extend or bend laterally, and an axial torque (AT) twists the spine about its long axis. (b) Analysis of forces during manual handling. A high tensile force (F) must be generated by the back muscles in order to lift up the weight (W), as well as upper body weight (w). The back muscles act on a short lever arm (d) relative to the centre of rotation (O), whereas W and w act on much longer lever arms (D and d w). Therefore F must be much greater than W. The equations show how to calculate the spinal compressive force (c) in a static analysis. F and C would increase greatly if the weight was lifted quickly. Reproduced from Adams et al. [15] with permission
3.2.2 Gravitational Loading
Superincumbent body weight exerts a vertical force of approximately 60 % of body weight on the lumbosacral joint. In upright postures, the gravitational force mostly compresses the spine, but also exerts a forward shear force on the lower lumbar vertebrae (Fig. 3.2a).
3.2.3 Inertial Forces During Rapid Movements and Falls
High forces are required to move the body quickly, in accordance with Newton’s 2nd Law of motion which states that

When a pilot ejects from an aircraft, the vertical force on his lumbar spine is equal to his upper body mass multiplied by the vertical acceleration (rate of change of velocity) of his seat. This acceleration can be sufficient to crush one or more vertebrae. More commonly, high inertial forces are generated by a fall on the buttocks, when the deceleration on impact magnifies the effect of upper body weight. Peak deceleration increases with the length of the persons’ legs and with the hardness of the landing.

(3.1)
3.2.4 Forces Arising from Muscle Tension
Muscle contractions pull their bony attachments towards each other, often compressing the joint that lies between them. Standing or sitting erect requires considerable ‘antagonistic’ muscle tension to stabilise the spine, in a similar manner to cables that stabilise a radio mast, so that the compressive force on the lumbar spine is typically 80–100 kg [25]. Muscle forces rise to high levels when objects are lifted on outstretched arms (Fig. 3.2b) because the external moment of the weight being lifted must be balanced by an internal moment, generated by muscle tension acting on a short lever arm, close to the centre of rotation. Internal muscle forces increase when movements are performed quickly [26, 27] because fast movements require high accelerations, which in turn require high forces (Eq. 3.1). It follows that maximum muscle forces are required to move the body as quickly as possible. Evidence from people suffering from epilepsy indicates that, when neural inhibitory controls are deficient, muscle tension can be high enough to crush a vertebra [28].
Confusion occasionally arises in medicolegal disputes (see final section, below) when an otherwise expert witness is unaware that forces generated by muscle tension and by falls can be much greater than the weight of the external object being handled and that the external object often weighs a good deal less than upper body weight, which must also be lifted against gravity by muscle tension (Fig. 3.2b).
3.3 Mechanisms of Disk Injury and Prolapse
3.3.1 Compressive Injury Causes Endplate Fracture
Excessive compressive loading, in the direction of the spine’s long axis, always damages a vertebral endplate before the adjacent disk. This fact has been demonstrated in many experiments on cadaveric spines [29] and probably contributed to the myth that healthy disks can degenerate but not become injured. The problem appears to have arisen historically when calculations first showed how great the compressive force on the spine can be (see Fig. 3.2b). Spinal loading became synonymous with spinal compressive loading, and the harmful effects of torsion and bending (see below) were overlooked.
Compressive failure occurs by fracture of the bony endplate, as the incompressible fluidlike disk nucleus causes it to bulge into the vertebral body [30]. Various types of endplate fracture have been described [29, 31], and later work showed how a wider range of vertebral body fracture patterns can occur, depending on posture (flexion or extension) and on the degree of disk degeneration [32]. If compressive loading is applied in a repetitive cyclic manner, in order to simulate manual labour, then similar fracture types are observed to those caused by sudden injury, but the peak compressive force can be up to 50 % lower [33]. Generally, it is the central region of the superior endplate (relative to the vertebra) that is damaged first, because it is the thinnest and supported by less dense trabecular bone [34]. Thickness and strength of the central endplate evidently have a very low margin of safety, probably because thickness is minimised to allow adequate metabolite transport into the disk nucleus [35].
3.3.2 Torsion and Bending Injuries Tear the Annulus
Axial rotation (‘torsion’) of the lumbar spine (Fig. 3.2a) occurs about a centre of rotation in the posterior quadrant of the disk [24]. The movement is resisted primarily by the anterior annulus and by the articular facets of the apophyseal joints. Damage probably occurs first in the apophyseal joint that is compressed, at a rotation angle of 1–3°, although this is not certain [24]. If the neural arch is removed, then unprotected lumbar disks can be rotated to much greater angles [36, 37], and failure occurs in the annulus, by a mechanism that involves delamination and rim tears [36]. However, torsion has not been shown to cause radial fissures or disk prolapse.
3.3.3 Complex Loading Can Cause Disk Herniation
Bending of the spine causes the intervertebral disks to be compressed on one side and stretched on the other (Fig. 3.3). In the case of forward bending (flexion), the posterior annulus is typically stretched by 50 % in a full range movement [38]. There is a corresponding decrease in annulus thickness in the radial (inner to outer) direction because the wet tissue must maintain constant volume, at least initially. Thinning of the posterior annulus in flexion has been confirmed in a radioactive tracer study [39], and it leaves this region of annulus vulnerable to injury from a high nucleus pressure. Consequently, if a cadaveric spine specimen is first positioned in full flexion or hyperflexion, and then compressed vigorously, the most common mode of failure is for the nucleus to herniate through the stretched posterior annulus [38, 40]. The herniated tissue (Figs. 3.4d and 3.5) emerges in a fraction of a second, sometimes with an audible ‘pop’. Spinal bending is crucial to the disk herniation mechanism, and no cadaveric experiment has created disk herniation in the absence of a high bending moment.




Fig. 3.3
The mechanism of disk prolapse depends on how the lumbar disks are deformed in flexed posture (such as that shown in Fig. 3.2b). The upper diagram shows typical dimensions of a lumbar disk in upright posture (AF annulus fibrosus, NP nucleus pulposus, H anterior height, h posterior height, T thickness of posterior annulus). Full flexion then deforms the annulus as shown in the lower diagram. Note that the posterior annulus typically is stretched vertically by 50 % and so must be thinned by 37 % to maintain constant volume. The stretched and thinned posterior annulus is then vulnerable to high pressures in the disk nucleus

Fig. 3.4
Structural defects in degenerated and herniated lumbar disks. Anterior is on the left in all figures. (a) Microradiograph of a midsagittal slice of a cadaveric vertebral body, showing a large Schmorl’s node resulting from an endplate fracture that was followed by vertical disk herniation. (b) Photograph of a cadaveric disk that has herniated vertically through the endplate in response to compressive loading. Lamellae of the inner annulus are collapsing into the decompressed nucleus. (c) Midsagittal section of a cadaveric intervertebral disk showing a complete radial fissure in the posterior annulus. (d) Similar to (c), except that in this specimen, high loading in bending and compression has caused the nucleus to herniate through the annulus. The disk was intact before loading. Images adapted from Adams et al. [15] with permission

Fig. 3.5
Large disk prolapse created by mechanical loading of a cadaveric spine in bending and compression (bar = 5 mm). The herniated tissue is mostly nucleus and inner annulus, but there is also some hyaline cartilage and bone from the adjacent endplate. Adapted from Adams et al. [15] with permission
The mechanism occurs most readily in spines aged 40–49 years [38] at which age there is a demonstrable fluid pressure in the nucleus [41], and yet the annulus is normally showing some signs of age-related weakening, most notably in the proliferation of concentric tears or clefts [1]. It is not easy to create disk herniation in this way if the disk is already degenerated [38] or if its nucleus has been dehydrated artificially by prior creep loading [42], indicating that high nucleus pressure plays an important role. Lower lumbar disks are more likely to herniate in this way [38], presumably because they have a thin posterior annulus which can be stretched substantially when the spine is flexed.
The underlying mechanism of herniation – pressurised nucleus pulposus bursting through a stretched and weakened annulus – has been clearly demonstrated by artificially raising the pressure inside animal disks and tracking the pattern of annulus disruption from inner to outer lamellae [43]. Later work emphasised the importance of flexion in causing a radial fissure to track through the posterior annulus, often close to its junction with the vertebral endplate [44]. Anterolateral bending (forwards and to one side) is closely related to disk prolapse in life [10], possibly because it causes maximum stretching of one posterolateral corner of the annulus, which lies further from the axis of bending than does the midline posterior annulus.
If the same combination of bending and compression is applied in a repetitive fashion, to simulate heavy manual labour, then a radial fissure (Fig. 3.4c) can be formed in a gradual progressive manner [45]. Only a small quantity of nucleus pulposus can then be extruded though the annulus, presumably because even a small loss of nucleus volume causes a relatively large drop in nucleus pressure [46] so that no more material is forced down the fissure. Experiments on young porcine disks confirm that this mechanism of progressive radial fissure formation in the posterior annulus does not require any prior degenerative changes in the disk [47, 48].
3.3.4 Endplate Involvement in Disk Prolapse
Disk herniations are mostly comprised of displaced annulus fibrosus and nucleus pulposus tissues [49], with the annulus/nucleus ratio increasing in patients aged over 30 years [50]. In addition, almost half of all herniations also contain some hyaline cartilage from the vertebral endplate [49, 51]. The relatively hard and smooth hyaline cartilage has a three-dimensional network of fine collagen type II fibrils which prevent it from swelling much, even when physically disrupted [52]. Consequently, hyaline cartilage fragments lose little of their proteoglycan content, so that they are relatively resistant to revascularisation, reinnervation and resorption [51]. This probably explains why their presence in a herniation is associated with more severe and prolonged clinical symptoms [50]. The cartilage endplate is mechanically integrated with the collagen network of the disk [53, 54], and yet it can easily be peeled off the underlying bone if the annulus is stretched vertically [51], so spinal bending is likely to play an important role in cartilaginous herniations. They are most frequent in patients aged 50–60 years, and relatively large cartilage fragments are most commonly found in herniations of L5–S1 disks [50].
Bone fragments also occur in some disk herniations [49], including those created in the laboratory [38]: see Fig. 3.5. The reported frequency in patients varies from 6 % [50] to 58 % [55], with the latter figure including any abnormality (including avulsion fracture) of the vertebral rim that could be visualised by CT. Close association between hyaline cartilage and bone fragments (Fig. 3.5) suggests that herniating annulus sometimes pulls away a small fragment of cartilage and bone endplate together. In other cases, displaced outer annulus appears to pull some bone off the posterior vertebral margin, without the inclusion of any hyaline cartilage. Detailed study of cadaveric bony endplates has shown that ‘erosions’ are common in the posterolateral margins of lower lumbar vertebrae [56]. Their appearance, location in the spine and association with patients’ symptoms are all consistent with them representing the stripping of cartilage off the underlying bone during disk herniation.
Defects in the human bony endplate are densely innervated [57], so it is no surprise that disk herniations involving the endplate are of particular clinical significance. As well as the above-mentioned link with prolonged sciatica, it appears that they are also associated with inflammatory (‘Modic’) changes in the endplate and with severe and painful disk infections [58, 59]. One possible explanation for these findings is that focal loss of hyaline cartilage endplate exposes the subchondral bone, which has much greater porosity and permeability than the cartilage [19]. Hence, focal cartilage loss increases focal endplate permeability, allowing anaerobic bacteria to enter the nucleus from the vertebral body and for inflammatory cytokines from disk cells to leave the nucleus and sensitise nerves in the bony endplate.
3.3.5 Mechanical Consequences of Disk Injury and Herniation
Endplate fracture creates more space for the disk nucleus (Fig. 3.4a, b), causing the pressure within it to fall immediately, often by more than 50 % [40, 60, 61]. Consequently, compressive load bearing is shifted from the nucleus to annulus and also from the disk overall to the neural arch [60], with effects being the greatest in older spines and at upper lumbar levels [61]. High stresses in the inner annulus can cause it to collapse into the decompressed nucleus, as shown in Fig. 3.4b [40]. Localised tears in the outer annulus have much less immediate effect on nucleus pressure [62], although animal experiments suggest that peripheral annulus tears can propagate inwards over several months until they reach the nucleus [5].
Another immediate mechanical consequence concerns the tissue displaced in a disk herniation: once removed from the pressurised confines of the disk, it can swell rapidly in tissue fluid. Cadaver experiments suggest that herniated tissue can more than double its weight in just 4 h and then shrink again during the following few days as it loses both proteoglycans and water [63]. These physico-chemical events could explain why some patients report a gradual onset of sciatica several hours after some recalled incident. Disk herniation also reduces nucleus pressure and volume, probably by an amount that is proportional to the herniated mass [64].
Over a longer timescale, nucleus decompression arising from either endplate fracture or disk herniation allows the annulus to bulge radially outwards, and also inwards, so that the disk loses height [65, 66]. Disk narrowing can result in the transfer of more than 50 % of the spinal compressive load on to the neural arch [20], where it appears to lead to osteoarthritis in the apophyseal joints [67–69]. Reduced separation of adjacent vertebrae creates slack in the intervertebral ligaments, so that they resist bending less [42], and the motion segment is then able to ‘wobble’ freely [70]. This ‘segmental instability’ can subsequently be reversed by the growth of vertebral body osteophytes [71].
3.4 Lumbar Disk Ageing and Degeneration
These processes are considered in detail elsewhere in this book, but they are mentioned briefly here in order to differentiate them from each other and to integrate them with the evidence concerning disk injury.
3.4.1 Inevitable Age-Related Changes in Human Lumbar Disks
All old intervertebral disks become dehydrated, discoloured and fibrous. Progressive age-related fragmentation and loss of proteoglycans explain why disk water content falls, especially in the nucleus [72]. Concurrent replacement of collagen type II with type I explains the increased fibrous texture of the nucleus and inner annulus [73], and increased collagen cross-linking involving sugars explains the yellowish discoloration [74]. These biochemical changes can, in turn, be attributed to an increasing proportion of disk cells becoming senescent [75] so that continuing repair (‘turnover’) of matrix macromolecules becomes slower with advancing age [76]. After growth is complete, age-related changes in disk composition make them stiffer, less able to distribute loading evenly on the adjacent vertebrae and more easily injured. Hence, minor structural defects tend to accumulate in the disk after the second decade [1]. In addition, the bony endplate becomes thinner and more porous with increasing age [77] reflecting systemic osteopaenia in many older people. However, most old disks do not become grossly disrupted or narrowed [78], and their internal mechanical functioning is little affected [41]. Generally, there is no blood vessel or nerve ingrowth in most old disks [79].
3.4.2 Features of Intervertebral Disk Degeneration
Disk ‘degeneration’ has traditionally been graded on numerical scales (e.g. 1–4) according to the presence or absence of specific features [80–82]. Features associated with ‘degeneration’ include accelerated age-related changes in composition, radial and circumferential fissures in the annulus, rim tears in the outer annulus, bulging or damaged endplates, annulus bulging radially outwards or collapsing inwards, disk space narrowing, marginal osteophytes on adjacent vertebral bodies and ingrowing blood vessels and nerves. Disk degeneration scales are exercises in pattern recognition and do not seek to define or explain what is going on. However, they can be used to establish statistical associations between disk degeneration and back pain [83, 84] and with aspects of abnormal disk function such as a decompressed nucleus [41], high stress gradients in the annulus [85] and decreasing spinal mobility [86].
3.4.3 What Is ‘Disk Degeneration’?
If all age-related changes in intervertebral disks are considered to represent ‘degeneration’, then it becomes difficult for epidemiologists to identify risk factors for the condition (because all disks age) or to assess the efficacy of disease-modifying treatments (reversing ageing may prove difficult!). Evidently, specific age-related changes that are closely related to pain need to be distinguished from ‘normal’ ageing.
The most widely cited definitions were suggested in 2006 [87]. Intervertebral disk degeneration is proposed to be ‘an aberrant, cell-mediated response to progressive structural failure’, and a degenerate disk is the one with ‘structural failure combined with accelerated or advanced signs of ageing’. Degenerative disk disease is ‘a degenerated disk which is also painful’.
The rationale behind these definitions is that some disks degenerate because they are so weakened by genetic inheritance, age and loading history that they can be injured during normal everyday activity. Disk injuries create regions of abnormally high and low matrix compressive stress [40], both of which inhibit matrix synthesis [88, 89] and create abnormal cell signalling [90], elevated enzyme activity [72, 91] and cell clustering [92] as the injured tissue attempts to repair itself. Unfortunately, repair is frustrated by the disk’s low cell density, so that degeneration often progresses to complete structural failure, as suggested in Fig. 3.6. The emphasis on structural failure is justified by the close association between structural defects and pain and by the fact that all of the structural features of disk degeneration can be created in cadaveric specimens by mechanical loading.
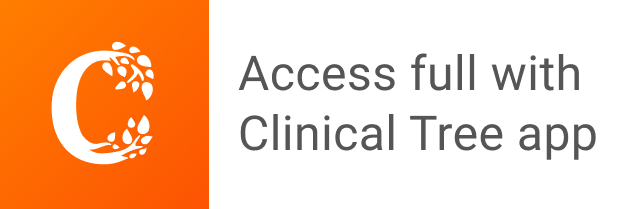