Fig. 17.1
Definitions of the knee, thigh, and hip (Adapted from Rupp et al. (2003). Effects of Hip Posture on the Frontal Impact Tolerance of the Human Hip Joint. Stapp Car Crash J 47:21–37. Used with permission of the Stapp Association)
Figure 17.2 illustrates the anatomy of the knee. The patella is a bone located over the anterior knee that primarily functions to increase the extension moment generated by the thigh musculature and protect the knee from anterior impacts. It is roughly triangular in shape, with a slight curve on the superior surface, where the quadriceps tendon inserts, and an apex at its inferior surface where the patellar ligament has its origin. The patella is mostly trabecular bone surrounded by a thin cortex. The majority of the posterior surface of the patella is articular cartilage that is divided in to lateral and medial portions by small central ridge. This ridge tends to translate along the intracondylar notch of the femur during normal knee flexion so the lateral and medial aspects of the retropatellar (i.e., posterior) surface contact the lateral and medial femoral condyles, respectively.
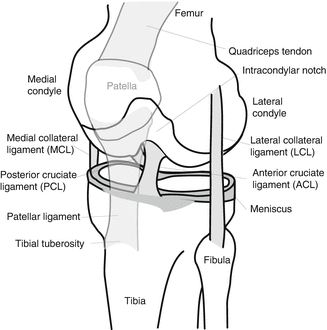
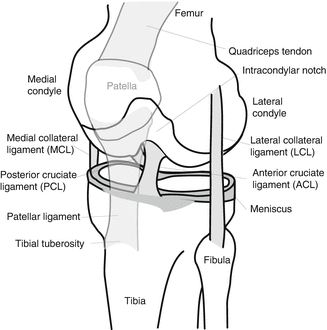
Fig. 17.2
Illustration of knee anatomy
The major ligaments of the knee include the posterior cruciate ligament (PCL), the anterior cruciate ligament (ACL), the medial collateral ligament (MCL) and the lateral collateral ligament (LCL). The PCL is composed of anterior and posterior bundles. The proximal attachment of the PCL is on the anterior-lateral portion of the medial femoral condyle, within the intracondylar notch and its distal attachment is on the posterior aspect of the medial tibial plateau. Because of this orientation the PCL provides the primary resistance to posterior translation of the tibia relative to the knee. The ACL is functionally described as having posterior-lateral and anterior-medial bundles, both of which have their proximal attachment in the posterior-medial aspect of the lateral femoral condyle within the intracondylar notch and have their distal attachment in the interspinous area of the anteriomedial tibia plateau (i.e., the superior tibia). Both bundles resist anterior displacement of the tibia relative to the femoral condyles with the anterior bundle providing more resistance when the knee is flexed and the posterior bundle providing more resistance when the knee is extended [2].
The MCL has its proximal attachment on the medial femoral epicondyle, near the adductor tubercle and its distal attachment on the lateral aspect of the tibial metaphysis below the knee joint, under the pes anserinus. The MCL provides primary resistance to valgus (lateral inward) bending of the knee. The LCL has its superior attachment to the lateral femoral epicondyle and its distal attachment to the fibular head. Its primary function is to resist varus (lateral outward) bending of the knee.
The femur is a long bone that, at its distal end, consists of medial and lateral condyles that articulate along the superior tibial plateau to form the knee joint. Proximal to the femoral condyles the femur narrows over the supracondylar region into a quasi cylindrical shaft that has a slight posterior to anterior curvature (Fig. 17.3). The proximal shaft of the femur widens into a subtrochanteric region followed by the trochanteric region, where many of the major muscles of the hip insert. The femoral neck arises from the trochanteric region, oblique to the femoral shaft. The medial femoral neck widens into a spherical femoral head, which rotates within the acetabulum of the pelvis. The acetabulum is the joint surface of the hip on the pelvis, where the illium, ischium, and pubis join. The hip is covered by a fibrous joint capsule that attaches to the rim of the acetabulum and the medial aspect of the trochanter (Fig. 17.4). The ischiofemoral, iliofemoral, and pubofemoral ligaments arise from the pelvis around the acetabulum and insert on the trochanter and act to stabilize the hip.
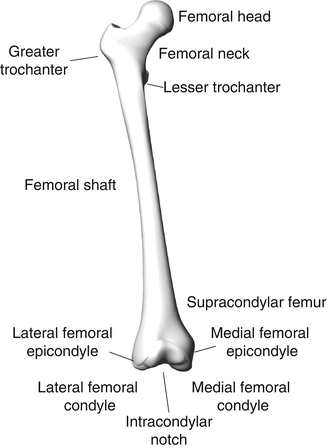
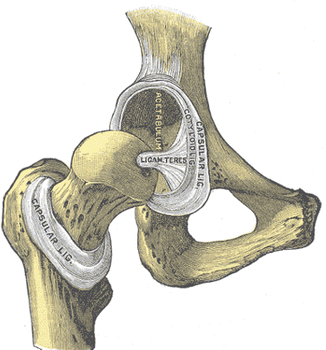
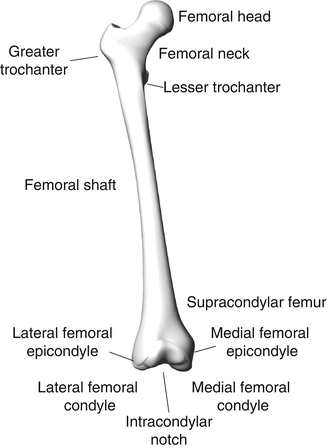
Fig. 17.3
Femur anatomy
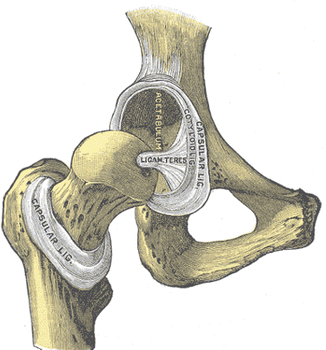
Fig. 17.4
Hip anatomy (From Gray’s Anatomy 20th Edition, 1916)
17.2 Types, Causes, and Mechanisms of Injury
17.2.1 Frontal Impact
KTH injuries to occupants in frontal motor-vehicle crashes typically occur from loading of the anterior surface of the flexed knee either by front-seat knee restraints or by interaction between the knees of rear seat passengers and the seat backs in front of them. This load is transferred from the patella and/or femoral condyles, through the femur and into the posterior acetabular surface of the pelvis. KTH injuries to occupants in frontal impacts with a lateral component may also occur from door or center console loading.
Figure 17.5 shows the distribution of types of KTH fractures and dislocations in frontal crashes. Knee injuries account for 16 % of all KTH injuries [3]. The most common type of knee injury is patellar fracture, which occurs when the patella is compressed against the femoral condyles. The likelihood of patellar fracture may be increased by tension in the patella caused by knee extensor muscle activation from pre-crash braking or bracing [4]. Knee impact with an angled surface such as knee bolster may also produce tension in the patella by causing it to slide superiorly, which places the patellar ligament in tension and is the likely cause of patellar ligament avulsion. Injuries to the posterior cruciate ligament (PCL) and other knee ligaments in frontal crashes are substantially less common than knee fracture, accounting for less than 1 % of moderate severity injuries in frontal crashes [5]. Injuries to the PCL and other knee ligaments may occur if loading is applied to the anterior tibia instead of the patella and femoral condyles [6] or may result from lacerations caused by interaction between the anterior knee and sharp surfaces.
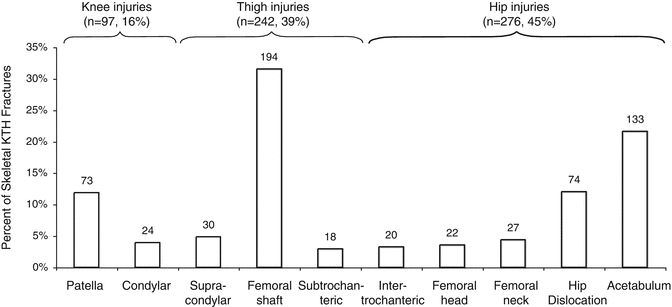
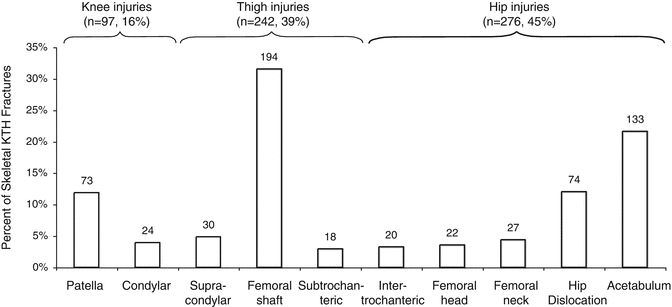
Fig. 17.5
Distribution of knee, thigh, and hip fractures and dislocations in frontal crashes UM CIREN from 1997 to 2003 (Adapted from Chang et al. [3])
Fractures of the femoral condyles typically occur because the patella is driven into the intracondylar notch [7] and, acting like a wedge, splits the condyles. This wedging action usually also causes a supracondylar femur fracture. Sup-racondylar femur fractures in the absence of a split condylar fracture are typically associated with a high-energy impact to the knee [8] and are typically highly comminuted.
Fractures to the thigh account for 39 % of KTH injuries [3] and are primarily femoral shaft fractures rather than intertrochanteric or supracondylar femur fractures. Femoral shaft fractures are usually due to axial compression. The curvature of the femoral shaft, with the midshaft lying both anterior and lateral to the line of action between the hip and knee, induces a posterior-to-anterior bending moment in the distal two-thirds of the femoral shaft under axial loading [9–11]. Knee loading that is preferentially applied to the medial femoral condyle rather than the lateral femoral condyle may also cause increased bending moments in the femur [12]. Because the center-of-pressure of the patella on the femoral condyles is below the midline of the femur when the knee is more flexed (i.e., the included angle between the tibia and femur is less than ~90°), loading of the flexed knee may also increase the likelihood of femoral shaft fracture by increasing posterior-to-anterior bending moment.
The mechanisms of trochanteric femur fractures from knee loading have not been extensively studied, because these injuries are not common. However, when these fractures do occur they are thought to involve tension in the region of the greater trochanter generated by axial loading through the femoral shaft, the support of the femoral head in the acetabulum, and the moment arm provided by the femoral neck [13]. Femoral neck fractures occur from a similar mechanism [10].
Hip fractures and dislocations account for 45 % of all KTH injuries in frontal crashes [3]. The most common hip injury is acetabular fracture, and in particular, fracture of the posterior acetabulum, although many different types of acetabular fractures can occur from knee loading [10, 14]. The pre-impact posture of the hip, changes in the posture of the hip during knee loading, and potential secondary loading paths from interaction between the trochanter and components of the seat or side door interior may affect hip fracture patterns in frontal crashes.
Morphologic differences in the acetabulum between men and women may also affect the acetabular fracture patterns and the likelihood of acetabular fracture [15, 16]. Specifically, women tend to have acetabular surfaces that, in a seated posture, cover a greater portion of the femoral head than men, and it has been hypothesized that stresses in the female acetabulum will be lower than those in the male acetabulum assuming similar femoral head size.
Body mass distribution also likely affects load transmission to the hip by changing the effective mass of the KTH complex and the amounts of body mass that become coupled to the KTH proximal and distal to the hip [17]. Body shapes that have a greater proportion of the flesh and torso mass proximal to the hip will likely see high forces transmitted to the hip and a greater likelihood for hip injury.
Body shape and body mass index (BMI) also alter the interaction between the seat belt and the pelvis such that a greater amount of flesh must be deformed before the lap belt can apply a restraint force to the pelvis. As a result, obese belted occupants have greater knee excursion and their knees contact knee restraints at higher velocities than normal BMI occupants [18, 19]. In addition to shifting the belt forward relative to the pelvis, the anterior body contours associated with obesity also tend to raise the belt above the pelvis and onto the abdomen [20].
17.2.2 Lateral Impact
KTH injuries to motor-vehicle occupants involved in lateral impacts occur from loading of the knee, thigh, and/or by the intruding door or side interior components as well as by lateral acceleration of the vehicle that drives the occupant into the door. Lateral impacts with a frontal component may also produce KTH injuries from loading of the anterior knee as described above. Loading of the pelvis by the seat belt and/or center console by the inboard hip are also hypothesized to increase the likelihood of pelvis injury.
Side impacts produce different patterns of lower-extremity injury patterns to occupants on the impacted side of the vehicle (nearside occupants) compared to occupants on the opposite side of the vehicle (farside occupants). For nearside occupants, approximately 70 % of AIS 2+ lower extremity skeletal injuries are to the hip/pelvis, 3 % are to the thigh, and 6 % are to the knee [21]. In contrast, for farside occupants, approximately 38 % of all AIS 2+ lower-extremity injuries are to the hip/pelvis, 5 % are to the thigh, and 17 % are to the knee [21]. Reasons for the differences in KTH injury patterns between farside and nearside occupants include that farside occupants receive less focal pelvic loading from vehicle side structures and components but have greater lateral movements of the leg-ankle-foot complex relative to the KTH. It should also be noted that most of the knee injuries to both farside and nearside occupants were AIS 2 (moderate severity) knee sprains, which have been considered minor injuries by other researchers and excluded from study [22, 23].
17.2.3 Pedestrian Impact
Knee-thigh-hip injuries from lateral impact to the lower extremities in a standing posture (i.e., pedestrian impact) typically occur from loading by the grill or the bumper of the vehicle, but can also result from impact by the hood or contact with the ground. Lower-extremity injuries represent approximately 26 % of all AIS 3+ pedestrian injuries [24] and 37 % of all AIS 2–4 injuries [25]. Approximately half of all AIS 2+ adult lower-extremity injuries in pedestrian impact are to the pelvis, femur, and knee [26]. For adults, approximately 65 % of all AIS 2+ KTH injuries in pedestrian impact are to the femur, 23 % are to the pelvis, and 12 % are to the knee [26]. Most of these injuries are skeletal fractures, although a meaningful proportion of knee injuries involve ligaments, most commonly the medial collateral ligament [27].
Ligamentous knee injuries in lateral impact typically occur from a combination of shear loading of the knee and lateral bending typically caused by impact to the leg, knee, or thigh. Fractures of the thigh are thought to occur from bending, while hip fractures occur from trochanteric loading, similar to lateral impacts to vehicle occupants.
17.3 Injury Tolerance and Biomechanical Response
17.3.1 Whole KTH
17.3.1.1 Injury Tolerance
The first biomechanical study of the tolerance of the KTH to frontal knee impact in an automotive crash environment was conducted by Patrick et al. [28]. In this study, an impact sled was used to decelerate seated whole embalmed cadavers using knee loading by lightly padded knee stops that were instrumented to measure forces applied to each knee. In tests of ten cadavers, fractures to knee, thigh, and hip (in different tests) were produced. The lowest fracture-producing force was 6.2 kN. Patrick et al. [29] later revised this tolerance value to 8.7 kN after an additional series of similar tests did not produce fractures at forces below 8.7 kN, and an analysis of posttest x-rays from their earlier study indicated that several of the subjects that sustained fractures at forces below 8.7 kN were osteoporotic.
Powell et al. [30] also measured the tolerance of the KTH by impacting the flexed knees of eight embalmed and two unembalmed stationary cadavers with a rigid flat-faced 15.6-kg ballistic pendulum. A repetitive test methodology was used where each cadaver knee was impacted at successively increasing impact velocities until fracture was produced. Fracture-producing impact speeds varied from approximately 5–9 m/s and the resulting knee loading rates, where reported, were above 4,000 N/ms. Injuries produced in this study were primarily to the knee and supracondylar femur, and the average fracture force associated with these injuries was 10 kN.
Melvin et al. [31] and Melvin and Stalnaker [8] recognized that much of the earlier KTH tolerance data were from tests on embalmed cadavers and were, therefore, of questionable value because of changes in skeletal mechanical properties caused by embalming. Melvin and Stalnaker therefore performed 31 impacts to the flexed knees of 14 unembalmed cadavers using an 11-kg flat-faced ballistic mass with varying amounts of padding on the impact surface. These tests almost exclusively resulted in knee and supracondylar femur fractures. Fracture-producing impact speeds were generally above 11 m/s and the associated knee loading rates, when published, were above 5,000 N/ms. The average fracture force from these tests was 18.4 kN, and no fractures were observed at forces below 13.4 kN.
The high incidence of knee and distal femur injuries coupled with the low incidence of hip fractures in early biomechanical studies [8, 29–31] were thought to indicate that the tolerance of the hip to anterior knee impact loading is greater than that of the thigh or knee and that the distal femur was the weakest part of the KTH complex. The dynamic tolerance of the distal femur was, therefore, considered to be an appropriate injury criterion for protecting the entire KTH complex. Consequently, distal femur and knee fracture force data collected from the dynamic knee loading performed by Melvin et al., Patrick et al., and Powell et al. [29–31] were used to establish a maximum force criterion of 10 kN for use in frontal crash testing. This criterion was implemented in Federal Motor Vehicle Safety Standard (FMVSS) 208, which currently requires that the peak force measured at the distal shaft femur of a midsize-male Hybrid III ATD not exceed 10 kN in various types of staged crash tests. The 10-kN maximum femur force criterion was later correlated to a 35 % risk of fracture or dislocation to the KTH complex [32].
Responding to concerns that real-world frontal crashes in the late 1970s were resulting in a significant number of injuries to the midshaft femur, proximal femur, and hip while the existing body of KTH tolerance data consisted almost exclusively of distal femur and knee injuries and few hip injuries, Melvin and Nusholtz [33] conducted a series of sled tests in which six whole unembalmed cadavers were decelerated by contact with padded instrumented knee stops. It was hypothesized that the longer knee loading durations and more realistic occupant kinematics provided by decelerating a moving body by applying force to the knees would be more likely to produce proximal femur and hip injuries. Two of the cadavers used in these sled tests were osteoporotic and consequently, data from these tests were not used in their analyses. In the remaining four tests, injuries to the knee, thigh, and hip were produced at peak applied forces that ranged from 10.4 to 25.6 kN. Because this range is similar to the range of peak applied forces associated with distal femur and knee fracture in the pendulum impact testing performed by Melvin and Stalnaker [8], the researchers concluded that the longer loading durations resulting from whole-body impact testing are associated with injuries to the femoral shaft, proximal femur, and hip.
Viano and Stalnaker [9] and Stalnaker et al. [34] impacted the knees of 12 seated unembalmed cadavers using an 11-kg flat-faced rigid ballistic mass. In these tests, femurs were denuded to allow for optical measurement of femoral deformation and knee impact velocities varied from 12 to 14 m/s. Contrary to the results of other pendulum-knee impact studies, these studies produced injuries to the femoral shaft and proximal femur at forces of 6–10 kN in addition to injuries to the distal femur and knee. Based on observations of the deformation of the femur, the primary mode of femur failure for knee impact loading was determined to be femur bending caused by loading of the anterior surface of the knee along with the anatomic curvature of the femoral shaft and the moment arm created by the femoral neck. One possible reason that femoral shaft and proximal femur fractures were produced in the Viano and Stalnaker study [9], but not in other pendulum-impact studies, is that the Viano and Stalnaker tests were conducted with a knee posture that was flexed so that the included angle between the tibia and the femur was approximately 45° (knee highly flexed). By loading the knee in this posture, force was applied to the posterior portion of the femoral condyles (below the midline of the femur), which would increase the inferior-superior bending moment on the femur and thereby increase the likelihood of femur fracture in bending.
Although a substantial amount of research has been done to quantify the tolerance of the whole KTH complex to knee impact loading and the tolerances of components of the KTH complex, few studies have explored load transmission from the knee to the hip and its effect of the risks of knee, thigh, and hip injuries. Horsch and Patrick [35] unilaterally impacted the knees of six whole cadavers, whole lower extremity (femur and all distal anatomy) specimens, and lower extremity specimens that were sectioned and potted at the mid-femur. In each case, impacts were performed using a flat-faced ballistic pendulum of varying mass at different velocities. Knee impact forces and femur and tibia accelerations from these tests have been used to define knee impact response specifications for crash test dummies and have demonstrated that loosely coupled mass in the lower extremities affects knee impact response.
Donnelly and Roberts [36] impacted the flexed knees of nine unembalmed cadavers using a rigid flat-faced 56-kg pendulum. A series of impacts at successively increasing velocity was performed on each subject until a knee, thigh, or hip fracture occurred. Knee impact forces applied to cadaver knees were measured and compared. In addition, a load cell was implanted in the distal femur of one KTH complex so that the axial force internal to the cadaver femur could be measured. Similar to earlier biomechanical studies, the rigid impacts to cadaver knees produced primarily knee and distal femur fractures and few femoral shaft or proximal femur fractures.
Peak axial forces measured by the implanted femur load cells were, on average, 53 % of the peak forces applied to the cadaver knees. The decreases in force between the knee and the location of the femur load cell were attributed to acceleration of the mass between the knee and femur load cell. Because the mass of the femur load cell and associated potting material was substantially larger than the tissue that it replaced, the drop in force between the knee and the femur load cell observed by Donnelly and Roberts was likely larger than that which would be observed in a cadaver without a femur load cell.
Rupp et al. [23] reviewed the results of earlier biomechanical studies and noted that most knee and supracondylar femur fractures were associated with high-rate knee impacts (2,000–5,000 N/ms loading rates) where fracture occurred within 5-ms after the start of impact. Rupp [13] also noted that the limited number of femoral shaft and hip injuries tended to occur in sled tests and under padded knee loading, both of which are associated with longer loading durations and lower loading rates. The association between higher knee loading rates and knee and distal femur fractures, coupled with the finding that there is a 2–5-ms lag between the start of loading of the knee and the start of cadaver pelvis acceleration [34] led Rupp et al. [23] to propose that laxity in the cadaver hip joint, which would prevent the development of a reaction force at the hip until it was removed was the primary reason that high-rate knee loading did not produce hip and proximal femur fractures.
Rupp et al. [17] further explored the response of the body to knee impact and force transmission from the knee to the hip using a combination of physical testing and lumped parameter computational modeling. Multiple knee impact tests were conducted using five unembalmed male cadavers that were approximately midsize in both stature and mass based on U.S. anthropometric data. In these tests, the knees were symmetrically impacted using loading conditions that spanned the 5th–95th percentiles of those observed in FMVSS 208 and NCAP tests (Hybrid III femur forces between 1.3 and 7.3 kN and femur loading rates between 100 and 1,000 N/ms). In addition, each cadaver was impacted after the connection between the thigh flesh and pelvis was cut, after the thigh flesh was removed, and after the torso was removed to provide data on the effects of these components on knee impact response.
Data from these tests and results of other studies were used with data on body segment masses to develop and validate a one-dimensional lumped-parameter model of the seated cadaver. Simulations of the whole body cadaver tests performed with this model predict that approximately 54 % of the peak force applied to the knee is transmitted to the hip and that this percentage is relatively constant over the range of knee impact conditions that are of interest for injury assessment in frontal crashes. Simulation results also confirm the hypothesis that high-rate, short-duration knee loading by a rigid surface is more likely to produce knee/distal femur fractures and less likely to produce hip fractures due to laxity in the hip that delays recruitment of pelvis mass and the development of fracture-level forces at the hip until after the fracture tolerance of the knee/femur has been exceeded.
17.3.1.2 Effects of Muscle Tension on KTH Injury
Existing biomechanical tolerance data and knee impact response studies with cadavers have produced almost exclusively knee and distal femur injuries and hip injuries, and few femoral shaft fractures. In contrast, data from frontal crashes collected by the Crash Injury Research and Engineering Network (CIREN) demonstrate that approximately one third of KTH injuries in frontal crashes are to the midshaft of the femur [13]. Chang et al. [3] hypothesized that the differences between real-world and laboratory KTH injury patterns are in part due to muscle activation in crashes from occupant braking/bracing that cannot be reasonably reproduced in cadaver tests. Specifically, Chang et al. [3] proposed that muscle tension increases flesh-to-skeletal mass coupling in the KTH and applies a pre-load to the femoral shaft and that the combination of these factors changes force transmission from the knee to the hip and increases the bending moment in the femoral shaft such that the risk of femoral shaft fracture is increased relative to the risks of other KTH injuries.
To test this hypothesis, Chang et al. [3] developed and validated a new finite element (FE) model that included detailed lower-extremity musculature and that tied muscle mass to force-generating elements so that the effects of muscle tension on flesh-to-skeletal mass coupling could be simulated. Activation of major thigh flexors and extensors using forces reported in the literature for bracing increased flesh-to-skeletal mass coupling and thereby increased the peak knee impact force relative to that produced without muscle tension for symmetric knee impact loading. Muscle tension also increased the percentage drop in force between the knee and the hip by preferentially increasing the mass coupled to the KTH distal to the hip and increased bending moments at the mid shaft of the femur. These results suggest that lower-extremity muscle tension tends to increase the potential for mid-shaft femur fractures relative to the potential for hip injuries.
Chang et al. [37] addressed some of the shortcomings of their previous work by developing improved estimates of lower extremity muscle activation for maximal braking and bracing exertions and applying these to estimates to the lower extremities of their FE model. Results of symmetric knee-impact simulations with this model using the improved muscle activation data supported the previous finding that muscle activation increases the drop in force between the knee and the hip. However, simulation results also demonstrated the reduction in the magnitude of force transmitted from the knee to the hip for a given knee impact condition was modulated by the increased force at the knee and by increased compressive forces at the hip due to activation of lower-extremity muscles. As a result, activation of lower-extremity muscle tension is not thought to substantially affect the risk of hip injuries. However, maximal muscle activation substantially increases the bending moments in the femoral shaft, thereby increasing the risk of femoral shaft from knee loading fractures from knee loading by 20–40 %.
17.3.1.3 Risk Curves
Injury risk curves for the skeletal knee-thigh-hip (KTH) complex have been developed that describe the probability of KTH fracture as a function of force applied to the anterior surface of the flexed knee. Morgan et al. [32] fit a Weibull distribution to peak knee-impact force data from 34 KTH fracture-producing tests from 6 studies in the National Highway Traffic Safety Administration (NHTSA) biomechanics database [22, 26, 38–41].
Kuppa et al. [22] recognized that Morgan et al. [32] excluded data from tests in which injury was not produced and that this biased the Morgan et al. risk curve. To address this shortcoming, Kuppa et al. used logistic regression to reanalyze peak knee impact force data from injury-producing and non injury-producing knee impacts in the six studies from which Morgan et al. obtained their data. Separate risk curves were developed for AIS 2+ and AIS 3+ injuries (Eqs. 17.1 and 17.2, respectively). However, because the AIS 3+ KTH injuries in the Morgan et al. dataset were almost exclusively distal femur fractures, the Kuppa et al. AIS 3+ risk curve is generally accepted to apply to the distal femur and not other parts of the KTH. The Kuppa et al. [22] risk curves were applied to the Hybrid III midsize male ATD and used to assess injury risk in frontal crash tests by the National Highway Traffic Safety Administration [42]. Injury risk curves for the small female ATD were developed using scaling [42, 43]. Fracture forces analyzed by Kuppa were not normalized to account for differences in response that are expected with varying subject size. Therefore, the Kuppa et al. risk curves are most applicable to vehicle occupants with the mean cadaver age and mass in the dataset Kuppa analyzed, which are 63 years and 69 kg, respectively.
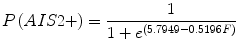
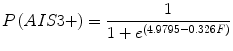
where F is peak force (kN) applied to the human knee or peak force at the Hybrid III femur load cell.
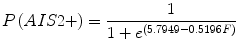
(17.1)
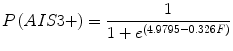
(17.2)
Laituri et al. [44] noted that the Kuppa et al. risk curves do not account for subject age and are not based on all available data. Therefore, they developed a new set of KTH risk curves for AIS 2+ injuries (Eq. 17.3). The dataset the Laituri et al. used to develop risk curves was limited to knee impacts performed with padded surfaces. Survival analysis was used to fit a Weibull distribution to the peak force data and to account for censoring (i.e., the occurrence of fracture at an unknown time prior to the time of peak force or the failure of a test to produce injury) in the dataset. The effect of cadaver age on fracture force was accounted for by restricting the analysis to peak forces from tests in which the subjects were older (age 60+) and by scaling the shape parameter of the resulting Weibull distribution (i.e., the risk curve) using relationships between age group and the tolerance of the femoral shaft to axial compression reported by Aoji et al. [45]. Prior to analysis, all peak-force data were normalized to account for the assumed effects of differences in subject size on fracture force using a dimensional analysis technique called equal-stress equal-velocity scaling [46]. Risk curves for females and different sizes of male occupants were developed using similar scaling techniques.
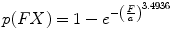
where
is peak force in kN applied to the knee normalized to a midsize male reference mass (75 kg) using equal-stress equal velocity scaling and a is an age scaling parameter that is equal to 15.9499 for ages 15–39, 15.0971 for ages 40–59, and 13.5495 for ages 60+.
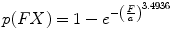
(17.3)

Rupp et al. [47] noted that Laituri et al. [44] used a limited dataset along with scaling to account for age and gender/stature effects rather than using the large amount of empirical data on KTH fracture force to define these effects. Rupp et al. therefore developed a new KTH risk curve for the whole KTH that was parameterized to account for the effects of subject characteristics and test conditions on KTH fracture forces and that appropriately accounted for censoring in peak knee impact force data (Eq. 17.4). The parametric KTH injury risk curve was used to define new KTH injury risk curves that apply to the adult small female and midsize male. Figure 17.6 compares the KTH risk curves developed by Kuppa et al., Laituri et al. and Rupp et al.
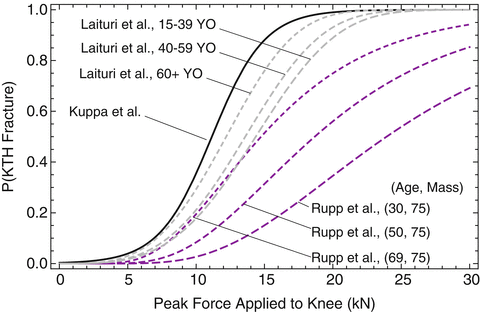
![$$ p(FX)=\varPhi \left[\frac{Ln\left[F\right]-\left(0.0081m-0.0124a+b\right)}{0.4519}\right] $$](/wp-content/uploads/2016/09/A31137_3_En_17_Chapter_Equ4.gif)
where,
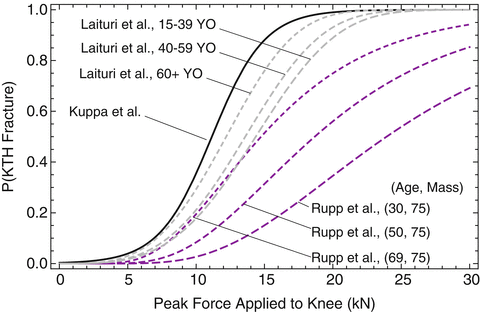
![$$ p(FX)=\varPhi \left[\frac{Ln\left[F\right]-\left(0.0081m-0.0124a+b\right)}{0.4519}\right] $$](/wp-content/uploads/2016/09/A31137_3_En_17_Chapter_Equ4.gif)
(17.4)
Φ is the cumulative distribution function of the standard normal distribution,
F is peak force applied to the knee in kN,
m is mass in kg,
a is age in years, and
b is 2.6224 for rigid and 2.9396 for padded knee impacts.
The risk curves described above relate force applied to the knee to the probability of any KTH fracture. However, because all of these risk curves are based primarily on injury data from studies that produced almost exclusively knee and distal femur fractures, these risk curves primarily apply to the knee and distal femur and are not applicable to the hip [10, 13].
Risk curves that apply to the entire KTH have traditionally been applied to peak axial compressive force measurements made by load cells located in the distal third of a crash test dummy femur. However, multiple studies have found that crash test dummies and cadavers produce different knee impact forces under similar knee loading conditions. Early studies found that knee impact forces produced by dummies were substantially higher than those produced by cadavers and it was therefore assumed that evaluating the probability of KTH injury using dummy femur load cell data and injury criteria based on cadaver knee impact force was a conservative approach [35, 36]. However, later work demonstrated that the differences between cadaver and dummy knee impact forces depend on the force-deflection characteristics of the surface loading the knee [13, 17, 48]. For impacts with a surface with constant stiffness, current dummies will over estimate KTH risk while for impacts with surfaces that are force limiting (e.g., crushable aluminum honeycomb) current dummies can under estimate KTH injury risk [13, 17, 48]. Ongoing development of the THOR, which is the next generation of the adult frontal-impact crash test dummy, should address these issues by improving the knee impact force biofidelity of the KTH complex [49].
17.3.2 Hip Injury
17.3.2.1 Frontal Impact
In real-world frontal crashes, the left hip is much more frequently injured than the right hip when the crash dynamics tend to move the occupant forward and to the left, while right hip injuries are more frequent in crashes where the occupant moves forward and to the right [10]. The KTH on the side of the body to which the occupant tends to move in a frontal crash will generally experience higher forces than the contralateral knee and lateral motion of the occupant’s pelvis induces adduction of the thigh (i.e., inward rotation of the hip). Hip adduction decreases the contact area between the femoral head and the acetabular surface and thereby decreases the force required to cause hip fracture [14].
Yoganandan et al. [50] first explored the effects of knee loading rate and thigh angle on KTH fracture tolerance using data from impacts to the anterior surfaces of the flexed knees of six whole unembalmed seated cadavers. These tests were performed using a 23.4-kg rigid flat-faced pendulum that impacted a cadaver knee at speeds between 4.3 and 7.6 m/s. A compressive preload was applied to the KTH complex to simulate muscle tension by a loop of seatbelt webbing wrapped around the knees and fastened behind the subject’s pelvis. A paired test methodology was used where one KTH complex from each subject was impacted so that the direction of applied force was approximately aligned with the long axis of the femur. The contralateral KTH complex was subsequently tested with the thigh either adducted/abducted and/or flexed from the direction of applied force. Results of these tests suggested that acetabular fractures are more likely with combined flexion and adduction, but no association between thigh angle and the force required to cause acetabular fracture could be ascertained because of the small sample size and large range of postures tested.
Rupp et al. [23] quantified the fracture tolerance of the cadaver KTH complex to loading applied to knee loading using a setup in which the pelvis was fixed to ensure that the force applied to the knee was the same as the force transmitted to the hip. All of these fixed-pelvis tests were conducted with the pelvis and thigh oriented in a typical automotive seated posture [51]. Knee loading was applied through an interface that was custom molded to the shape of each subject’s knee to ensure that applied forces were distributed over the entire anterior surface of the knee. All tests produced posterior acetabular fractures and the distributions of fracture types that occurred in these tests matched those observed in real-world frontal crashes from the University of Michigan CIREN database, suggesting that the fixed pelvis boundary condition did not affect test results beyond eliminating the inertially induced decrease in force transmitted from the knee to the hip. The average acetabular fracture tolerance from these tests was 5.7 kN (SD = 1.4 kN).
Rupp et al. [23] also measured the fracture tolerance of the uninjured knee/femur specimens that were used in the fixed-pelvis tests by supporting the femoral head in an “acetabular cup” and applying a force to the knee. All of these knee/femur tolerance tests produced femoral neck fractures, and the average fracture tolerance from these tests was 7.6 kN (SD = 1.6 kN). When considered together with the results of previous studies, the injury patterns and fracture forces measured in the fixed-pelvis and fixed-femoral-head tests demonstrate that the acetabulum is the weakest part of the KTH complex for axial knee impacts in typical automotive postures, and that the femoral neck has the next lowest tolerance to distributed knee impact loading.
Rupp et al. [10] quantified the effects of hip posture on hip tolerance using similar fixed-pelvis test methods as Rupp et al. [23] but with a larger sample size. A paired comparison test methodology was used in which one hip from each subject was tested in a standard automotive seated posture and the contralateral hip was tested in a posture that was either ab-/ad-ducted and/or flexed from this posture. When data from these tests were combined with data from Rupp et al. [23], the average fracture tolerance of the hip in the neutral posture was 6.1 ± 1.5 kN. Hip tolerance decreased by an average of 34 ± 4 % with 30° of flexion from the neutral posture and by 18 ± 8 % with 10° of adduction from the neutral posture. Subsequent fixed-pelvis testing reported by Rupp [13] demonstrated that 15° of hip abduction increased hip tolerance by approximately 15 % and that the effects of hip posture on tolerance are generally linear with posture. Isolated hip tolerance testing by Bass et al. [52] conducted using similar methods to those of Rupp et al. [10] confirm the effects of hip posture on hip injury tolerance.
Rupp et al. [53] applied survival analysis to fracture force data from 27 hip tolerance tests conducted with the hip oriented in a standard automotive seated posture and developed a risk curve that was parametric with specimen stature (which was the only parameter among age, gender, body mass index, bone mineral density, and stature that was a significant predictor of fracture force). Based on data from Rupp et al. [13], this risk curve was modified so the mean shifted depending on the amount of hip flexion or abduction. Equation 17.5 and Fig. 17.7 show this risk curve. Typical values of flexion and abduction used with this risk curve are 30° and 15°, which represent typical amounts of peak hip flexion and hip abduction from a standard automotive seated posture that are present in a frontal crash at the time of peak femur force.
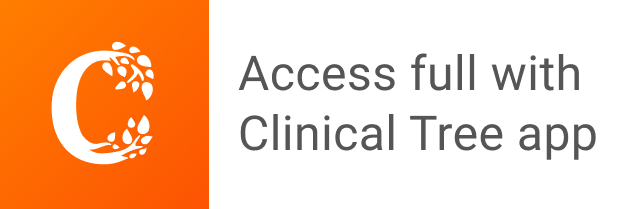