Kinematics of the Hip
Stephen J. Huffaker
Tsung-Yuan Tsai
Harry E. Rubash
Guoan Li
Young-Min Kwon
The term “kinematics” comes from the Greek root noun kinema, motion, and verb kinein, to move, to describe a branch of mechanics related to the motion of objects without concern for mass or outside forces. In contemporary orthopedics, kinematics has come to reflect the investigation of a joint’s mechanical properties throughout motion. This involves the stresses that are seen by the joint throughout motion, the limits of and to motion, and the interaction of these two during joint function. This chapter will discuss these properties with regard to the hip joint. It is important to note that much of the previous half century of arthroplasty research into longevity of implants has focused on the material science of prostheses and has led to the advent of highly specialized materials that are resilient to millions of cycles translating to decades of function for patients. Though continued endeavors and improvements to arthroplasty materials are of value in further improving the longevity of implants, component positioning and dynamic function are being realized as a significant factor in total hip arthroplasty stability and lifespan. As such, an appreciation and understanding of hip kinematics, including of the native hip, the diseased hip, and the replaced hip, is critical for the next stage of improvements to hip arthroplasty. This chapter will outline and discuss the current understanding of how hip kinematics change throughout common pathologies as well as following hip arthroplasty.
Fundamentals of Hip Joint Motion
The hip or acetabulofemoral joint (articulatio coxae) is the connection between the femur and acetabulum of the pelvis. The hip represents the conduit through which the weight of the head, arms, and thorax are transmitted to the lower extremities. In this role, the hip must be able to dynamically carry and transmit both the gravitational forces of the body and the ground reaction forces of the lower extremity throughout a large range of positions. This is accomplished through a combination of both static and dynamic stabilizers that help to prevent joint dislocation and to maintain mechanical efficiency. The hip must, at the same time, be highly dynamic, allowing for a large degree of motion to provide the necessary movements required for the wide range of activities that are demanded of the human body.
The Role of the Hip
While standing, the entire weight of the upper body is transmitted through the lumbar spine and transferred to the sacrum and across the sacroiliac joint to the ilia. This weight is then transferred to the acetabula and the neck of the femur while standing. Consequently, the hip’s most important role is in maintaining balance and stability of the body in an erect position. The following sections will discuss the structural factors contributing to the balance of stability and mobility of the hip.
Stability
Osteology
The acetabulum lies on the lateral aspects of the inferior pelvis and is resultant from the triradiate cartilage growth center of the pelvic innominate bone at the union of the ilium, ischium, and pubic bones. Though the acetabular recession or cup appears to have a hemispherical shape, it is only spherical in nature in the upper one-third or dome, allowing for maximal distribution of force in areas required for weight bearing during upright stance. As previously mentioned, the acetabulum inclined giving an acetabular index (slope of the acetabulum on an AP radiograph) an average of 38 degrees in males and 40 degrees in females (1) allowing for increased abduction yet decreased adduction (Fig. 11.1A). In the sagittal plane, the acetabulum is anteverted an average of 16 degrees for men and 19 degrees for women (2) allowing for increased flexion arc as well as posterior coverage and preventing escape of the head during deep flexion.
On the femoral side of the joint, the osseous structure also contributes to joint stability during both dynamic and static loading. The femoral head is semispherical in shape, ranging from hemispherical to two-thirds of a complete sphere. The head quickly tapers into the femoral neck that joins the remaining femur. The distance between the center of the femoral head and the anatomic axis of the femur represents the “head/neck offset” and is directly related to the moment arm and efficiency of the hip abductors. The orientation of the femoral head/neck complex with regard to the femoral epicondylar axis also plays a critical role in the stability of the hip during static and dynamic hip functions. Similar to the anteversion of the acetabulum, the femoral head/neck is anteverted with regard to the anatomic axis by
approximately 10 to 20 degrees in adults (Fig. 11.1B). This internal rotation of the femoral shaft in conjunction with the anteverted acetabulum allows for increased flexion arc of the hip, particularly with the leg in slight external rotation, a key component to the normal gait pattern as discussed later (3). The femoral head/neck is also angled cephalad in the coronal plane giving an overall head/neck angle of 125–140 degrees of valgus orientation (Fig. 11.1C). This allows for a more vertical transfer of the head, arm, and trunk weight through the acetabulum and down the femoral shaft while still maintaining adequate head/neck offset to allow the abductors to sufficient moment arm to function.
approximately 10 to 20 degrees in adults (Fig. 11.1B). This internal rotation of the femoral shaft in conjunction with the anteverted acetabulum allows for increased flexion arc of the hip, particularly with the leg in slight external rotation, a key component to the normal gait pattern as discussed later (3). The femoral head/neck is also angled cephalad in the coronal plane giving an overall head/neck angle of 125–140 degrees of valgus orientation (Fig. 11.1C). This allows for a more vertical transfer of the head, arm, and trunk weight through the acetabulum and down the femoral shaft while still maintaining adequate head/neck offset to allow the abductors to sufficient moment arm to function.
One of the most critical parts of the hip’s osseous structure is the articular incongruence. The acetabulum is only spherical in nature in the weight-bearing dome, whereas the femoral head is spherical in as much as two-thirds of the articular surface. This as well as the angular differences mentioned above (i.e., anteversion and inclination) results in a portion of the femoral head articular surface that is “uncovered” at any time. Maximal coverage of the femoral head, and therefore maximal congruence, is experienced in the flexed, abducted, and externally rotated position (FABER). However, during upright stance and throughout the gait cycle, the femoral head has the greatest amount of coverage during maximal force transmission (i.e., heel strike and single leg stance).
Ligamentous and Capsular Structures
Though the inherent stability of the hip’s spheroidal design imparts a great degree of stability to the joint, additional ligamentous support, consisting of extra- and intra-articular fibrous bands, act in concert as checkrein to all six degrees of hip motion. The extra-articular ligaments consist of band-like thickenings of the capsule that allow for motion within a defined arc. Cadaveric studies have demonstrated some variability and controversy regarding the exact nature and discrete nomenclature of these bands (4), however, classically the capsule is described as having four extracapsular ligaments consisting of longitudinal fibers of the iliofemoral, the ischiofemoral, and the pubofemoral ligaments as well as the annular fibers of the zona orbicularis. These ligaments play a crucial role throughout motion and at different joint positions as evidenced by the change in motion after sectioning (Table 11.1).
Of the extracapsular ligaments, the iliofemoral ligament confers the greatest amount of static stability during erect stance. The ligament emanates as two distinct bands from a small interval between the anterior inferior iliac spine (AIIS) and the anterior superior acetabular rim and joins to the anterior femoral neck base (Fig. 11.2) (5). This Y-shaped confluence of fibers was originally described by Henry Jacob Bigelow, giving rise to the eponymous term Y-Ligament of Bigelow and is the key static stabilizer of the hip during stance primarily resisting hyperextension though they also tighten
through external rotation, adduction, and anterior femoral head translation (Table 11.1) (4,5). Biomechanical studies have further demonstrated that the iliofemoral ligament has the greatest force to failure and overall stiffness of all the capsular ligaments, corresponding to approximately 350 N and 100 N/mm, respectively (6).
through external rotation, adduction, and anterior femoral head translation (Table 11.1) (4,5). Biomechanical studies have further demonstrated that the iliofemoral ligament has the greatest force to failure and overall stiffness of all the capsular ligaments, corresponding to approximately 350 N and 100 N/mm, respectively (6).
Table 11.1 Mean Internal and External Rotation After Release of Corresponding Ligament | ||||||||||||||||||||||||||||||||||||||||||||||||||||||||||||||||||||||||
---|---|---|---|---|---|---|---|---|---|---|---|---|---|---|---|---|---|---|---|---|---|---|---|---|---|---|---|---|---|---|---|---|---|---|---|---|---|---|---|---|---|---|---|---|---|---|---|---|---|---|---|---|---|---|---|---|---|---|---|---|---|---|---|---|---|---|---|---|---|---|---|---|
|
Along the anterior and inferior portions, the hip capsule contains a fibrous thickening termed the pubofemoral ligament or pubocapsular ligament (Fig. 11.2A). This band originates from a wide base along the anterior aspect of the superior ramus of the pubis and along the anterior obturator crest projecting toward the anterior, inferior intertrochanteric ridge. The pubofemoral ligament is critical in resisting lateral translation, excessive abduction, and external rotation (Table 11.1) (4,5).
The ischiofemoral ligament is the main posterior ligamentous thickening of the hip capsule and originates along the entirety of the posterior, superior acetabular rim of the ischium and attaching to the medial aspect of the anterosuperior greater trochanter (Fig. 11.2B). As these fibers reach the base of the femoral neck they become confluent with the deep arcuate fibers of the zona orbicularis. A second fibrous band travels from the ischium to the posteromedial base of intertrochanteric ridge (5). In total, the ischiofemoral ligaments provide primary support to resist internal rotation throughout hip motion, while the superior bands act as secondary stabilizers to adduction with the hip in flexion (4,6).
The posterior capsule is further bolstered by a deep arch of fibers that run transversely along the base of the femoral neck
and do not cross the hip joint. These fibers are collectively termed the arcuate ligament and interconnect the superomedial aspect of the greater trochanter and the superior rim of the posterior lesser trochanter (6). Though the greatest thickening of fibers lies posteriorly, the arcuate ligament is a part of a larger annular structure termed the zona orbicularis that encircles the entire femoral neck base (7,8). This added collection creates a retaining ring that both acts to resist extremes of flexion and extension as well as distraction forces (6,7).
and do not cross the hip joint. These fibers are collectively termed the arcuate ligament and interconnect the superomedial aspect of the greater trochanter and the superior rim of the posterior lesser trochanter (6). Though the greatest thickening of fibers lies posteriorly, the arcuate ligament is a part of a larger annular structure termed the zona orbicularis that encircles the entire femoral neck base (7,8). This added collection creates a retaining ring that both acts to resist extremes of flexion and extension as well as distraction forces (6,7).
In addition to the extracapsular ligaments, arguably one of the most critical static components of hip stability is the labroacetabular complex. This structure consists of an intracapsular fibrocartilaginous lip that encircles all but the inferomedial aspect of the acetabular rim acting to functionally deepen the acetabular cup. The labrum consequently has a gasket effect on the overall femoroacetabular articulation, effectively sealing the joint throughout the majority of the motion arc. This has a similar effect as the labrum in the glenohumeral joint and results in a negative pressure capsule that resists deformity both during loading and unloading (9). Moreover, the rate of load and the total displacement of force applied to the hip joint itself is directly related to the presence of an intact labral seal (9). Further discussion about the role of the labrum in joint forces will be discussed in future sections.
Dynamic Stabilizers
Dynamic stabilizers of the hip joint can be divided into flexors, extensors, internal and external rotators, abductors, and adductors. Similar to the muscles of the rotator cuff, antagonist function during the extremes of motion helps to maintain the femoral head within the acetabulum. However, in the hip, the interrelationship between muscle groups is much less well understood and less clearly coupled as compared with the muscles of the rotator cup. Conceptually, it is understood that opposing muscle groups help to counter each other’s motion to create hip rotation with minimal translation as previously mentioned. For example, as the hip abducts through gluteus minimus and medius function, the adductor complex eccentrically contracts to help translate the femoral head back into the center of the acetabulum, thereby maintaining concentric and primarily rotatory motion. However, as the insertion points on hip abductors and the abductor complex are not directly opposite on the femur, the resulting difference in lever arms and moments results in a more complex intermuscular relationship than, say, that of the subscapularis and infraspinatus. Moreover, additional dynamic stabilizers also participate in direct stabilization of the hip joint by acting as physical blocks to femoral head escape. In particular, muscles that pass directly over or even attach to the hip capsule may physically bar translation of the femoral head with applied loads. One example of this is the iliopsoas muscle during erect stance and at the extremes of extension. In this position the myotendinous portions directly overlay the anterior hip capsule such that as the muscle contracts it results in hip flexion as well as anterior translation force on the femoral head. However, the taut muscle overlying the anterior hip acts to buttress the anterior capsule against translation. Similar behaviors by the gluteus minimus and the indirect head of rectus femoris may also help to contribute to hip stability in early abduction and flexion, respectively.
Mobility
Active Mobility
Though the major mechanical changes experienced by the hip are rotational in nature, most motion of the lower extremity requires a combination of both translational and rotational changes in the hip joint. The exception to this is flexion/extension in neutral abduction and external rotation. In this position and throughout this arc of motion, the center of the femoral head and acetabulum are maintained in an overlapping position by evenly balanced forces of hip flexors and adductors (3,10,11). Outside of this arc or motion, rotation of the femoral head results in associated translation that must be countered by opposing static or dynamic stabilizers to maintain a reduced joint (11).
Range of Motion
Experimental averages for the normal range of motion of the native hip are provided in Table 11.2, however, the range of motion demanded of the hip through life’s activities is highly variable between patients. Regardless, a minimum amount of motion is required for activities of daily living such as sitting and standing, climbing stairs, or walking; and evaluation of one’s motion can allow for improved assessment of functional limitations. In general, Heller et al. (12) demonstrated that for walking unassisted on level ground the hip requires a much smaller arc of motion with a flexion/extension arc of 40 degrees, abduction/adduction arc of 10 degrees, and 10-degree rotational arc (Table 11.2). The addition of other activities such as uneven surface ambulation, running, rising from a chair, etc. require a much greater range of motion (13). In terms of where this motion, or more importantly, where the limits arise from, it is the osseous structures that determine the absolute limits of motion of the joint whereas the soft tissue components determine the realized or in vivo limits of motion.
Table 11.2 Normal Motion Ranges for the Adult Native Hip at Rest and Observed During Level Surface Gait | ||||||||||||||||||||
---|---|---|---|---|---|---|---|---|---|---|---|---|---|---|---|---|---|---|---|---|
|
The hip joint itself is constrained by the mechanical limitations of the bones that make up the joint in that at the extremes of motion there is osseous impingement. Although the osseous anatomy may change in response to pathology (i.e., osteoarthritis, trauma, osteonecrosis, etc.), it is the soft tissues (ligaments, muscles, labrum, etc.) that determine the
in vivo range of motion. Soft tissues act to either restrain motion or block motion by interposition and impingement. Turley et al. (3), investigated this phenomenon by comparing the extremes of motion experienced by the hips of subjects through a series of activities of daily living. They then compared these findings to the arc of motion that would be predicted by the osseous acetabulofemoral joint alone. In the overlap of these two motion arcs, they found a significantly decreased range of functional motion as compared with osseous motion particularly in extension and flexion with adduction (Fig. 11.3). Interestingly, this suggests that during extension, the hip is stabilized and limited primarily by soft tissues, not by osseous impingement as might be suggested by an anteverted acetabular cup. Conversely, Turley et al. (3) found that osseous impingement occurred in vivo at the extremes of adduction in an upright stance as well as abduction at 90 degrees of flexion corresponding to impingement of the lesser trochanter on the pubis and the greater trochanter on the lateral acetabular rim. This is particularly salient with regard to total hip replacement as implant or osseous impingement versus soft tissue impingement presumably puts the patient at greater risk of dislocation and early wear/failure (see Section “Hip Kinematics in Common Pathologies”).
in vivo range of motion. Soft tissues act to either restrain motion or block motion by interposition and impingement. Turley et al. (3), investigated this phenomenon by comparing the extremes of motion experienced by the hips of subjects through a series of activities of daily living. They then compared these findings to the arc of motion that would be predicted by the osseous acetabulofemoral joint alone. In the overlap of these two motion arcs, they found a significantly decreased range of functional motion as compared with osseous motion particularly in extension and flexion with adduction (Fig. 11.3). Interestingly, this suggests that during extension, the hip is stabilized and limited primarily by soft tissues, not by osseous impingement as might be suggested by an anteverted acetabular cup. Conversely, Turley et al. (3) found that osseous impingement occurred in vivo at the extremes of adduction in an upright stance as well as abduction at 90 degrees of flexion corresponding to impingement of the lesser trochanter on the pubis and the greater trochanter on the lateral acetabular rim. This is particularly salient with regard to total hip replacement as implant or osseous impingement versus soft tissue impingement presumably puts the patient at greater risk of dislocation and early wear/failure (see Section “Hip Kinematics in Common Pathologies”).
Function, ROM, and the Gait Cycle
The human gait cycle has long been studied through the use of high-speed cameras and observational studies particularly in the injured or weakened patient. With the advent of computer technology, these methods have been amended by surface mounted markers and subsequent computational models that allow for improved understanding of joint position and even muscle tone/forces throughout the gait cycle. It is beyond the scope of this chapter to delve into the intricacies of total gait mechanics but instead we will discuss the gait cycle and how the hip functions throughout.
The human gait comprises two main phases, stance phase corresponding to 60% of gait and swing phase making up the remainder. In its simplest form, stance phase is subdivided into additional subphases representing heel strike, flat foot, midstance/single leg stance, and pushoff. Each of these phases is named according to the position of the ipsilateral foot, and each phase quickly blends into the next through a transfer of energy and momentum as well as eccentric and concentric muscle loading (Fig. 11.4). Swing phase can be subdivided into an acceleration phase, where the leg moves to catch up with and pass the torso, midswing, and deceleration phase where the swinging leg moves past the torso and prepares for heel strike, the start of the next stride. Though this is a gross simplification of the stride pattern seen in humans, it serves as a blueprint for thinking about the position of the hip throughout the gait cycle and the points at which static and dynamic factors are involved.
With regard to the hip throughout this cycle, as mentioned previously, the main role is to support the weight of the body, transferring this force through the pelvis and into the lower extremity. However, it must do this through a dynamic range, relying on the combination of static and dynamic stabilizers to not only maintain concentric alignment of the joint but to help transition through the gait cycle and locomotion (Fig. 11.4). As the limb begins the gait cycle, the hip is flexed, slightly externally rotated and in neutral adduction centering the femoral head in the acetabulum and allowing for a large degree of femoral head coverage (Fig. 11.4). After heel strike, the hip extends as the gait progresses toward toe off. Just prior to toe off, the hip begins to flex and abduct to allow for the leg to swing through during the swing phase and clear the ground (Fig. 11.4). This is met with internal rotation through the acceleration phase followed again by external rotation at the end of swing phase to maintain a centered femoral head as the leg passes from behind the body to in front.
At any given point of the gait cycle, the forces seen by the hip are the sum of both moment forces produced by hip musculature and load forces distributed from the head, arms, and thorax. The net sum of these forces is the total amount of force seen across the joint, also known as joint reactive force. As the hip moves through the gait cycle, the direction and magnitude of this force vector changes, ranging from 3.5 to 5 times the subject’s total body weight (14). For example, as the hip moves through the swing phase the hip starts in extension and ends in maximal flexion relying
on the pull of hip flexors to do so. At the start of this process, the distal attachments of the hip flexors (i.e., the lesser trochanter, the quadriceps tendon, etc.) are posterior to the center of rotation of the hip causing a flexion moment as well as a superior and even anterior translation of the femoral head in the acetabulum (i.e., cephalad and anterior directed net force vector). As the cycle continues, the vector of pull by the hip flexors changes and becomes more posteriorly directed in the acetabular cup. The result is a rotation of the direction of joint reactive forces seen by the hip from anterosuperior to posteriorly directed (Fig. 11.5). As a result, the critical stabilizers against hip translation as well as load distribution must change accordingly, in this case protecting from anterior translation at the end of stance/early swing phases to preventing posterior translation at the end of swing phase/early heel strike. It is this careful interplay between concentrically contracting muscles and dynamic and static stabilizers that are dramatically altered in a number of pathologic conditions of the hip and following the surgical solutions to address them.
on the pull of hip flexors to do so. At the start of this process, the distal attachments of the hip flexors (i.e., the lesser trochanter, the quadriceps tendon, etc.) are posterior to the center of rotation of the hip causing a flexion moment as well as a superior and even anterior translation of the femoral head in the acetabulum (i.e., cephalad and anterior directed net force vector). As the cycle continues, the vector of pull by the hip flexors changes and becomes more posteriorly directed in the acetabular cup. The result is a rotation of the direction of joint reactive forces seen by the hip from anterosuperior to posteriorly directed (Fig. 11.5). As a result, the critical stabilizers against hip translation as well as load distribution must change accordingly, in this case protecting from anterior translation at the end of stance/early swing phases to preventing posterior translation at the end of swing phase/early heel strike. It is this careful interplay between concentrically contracting muscles and dynamic and static stabilizers that are dramatically altered in a number of pathologic conditions of the hip and following the surgical solutions to address them.
In Vivo Hip Kinematics
Though imaging modalities and diagnostic criteria of hip pathology are frequently based on static findings (i.e., radiographs, laboratory values, etc.), it is important to remember that all pathologies of the hip are dynamic in nature and that disease processes cannot occur without an eventual effect on motion. Moreover, although the past and current innovation for THA has been on improving fixation methods and bearing surfaces, the current challenges with the contemporary THA involve in vivo dynamic phenomenon such as edge loading, impingement, and dislocation. As such a kinematic
understanding of the hip is critical for evaluating the etiology of hip disease as well as in improving our ability to restore function through arthroplasty.
understanding of the hip is critical for evaluating the etiology of hip disease as well as in improving our ability to restore function through arthroplasty.
Local Coordinate System
The hip is a monoarthrodial spheroidal joint which comprises a near spherical femoral head and a complementary acetabular cup resulting in a very strong and stable multiaxial ball-and-socket synovial joint. In discussing the kinematics and biomechanics of the hip joint, it is important to establish the planes of motion in which the joint moves. The hip has motion with six degrees of freedom, meaning that it is able to rotate and translate along three axes. The rotational axes are described in terms of abduction/adduction or rotation along the anterior/posterior axis (x-axis), internal/external rotation or rotation along the cephalad/caudal axis (y-axis), and flexion/extension or rotation along the medial/lateral axis (z-axis) (Fig. 11.6) (15
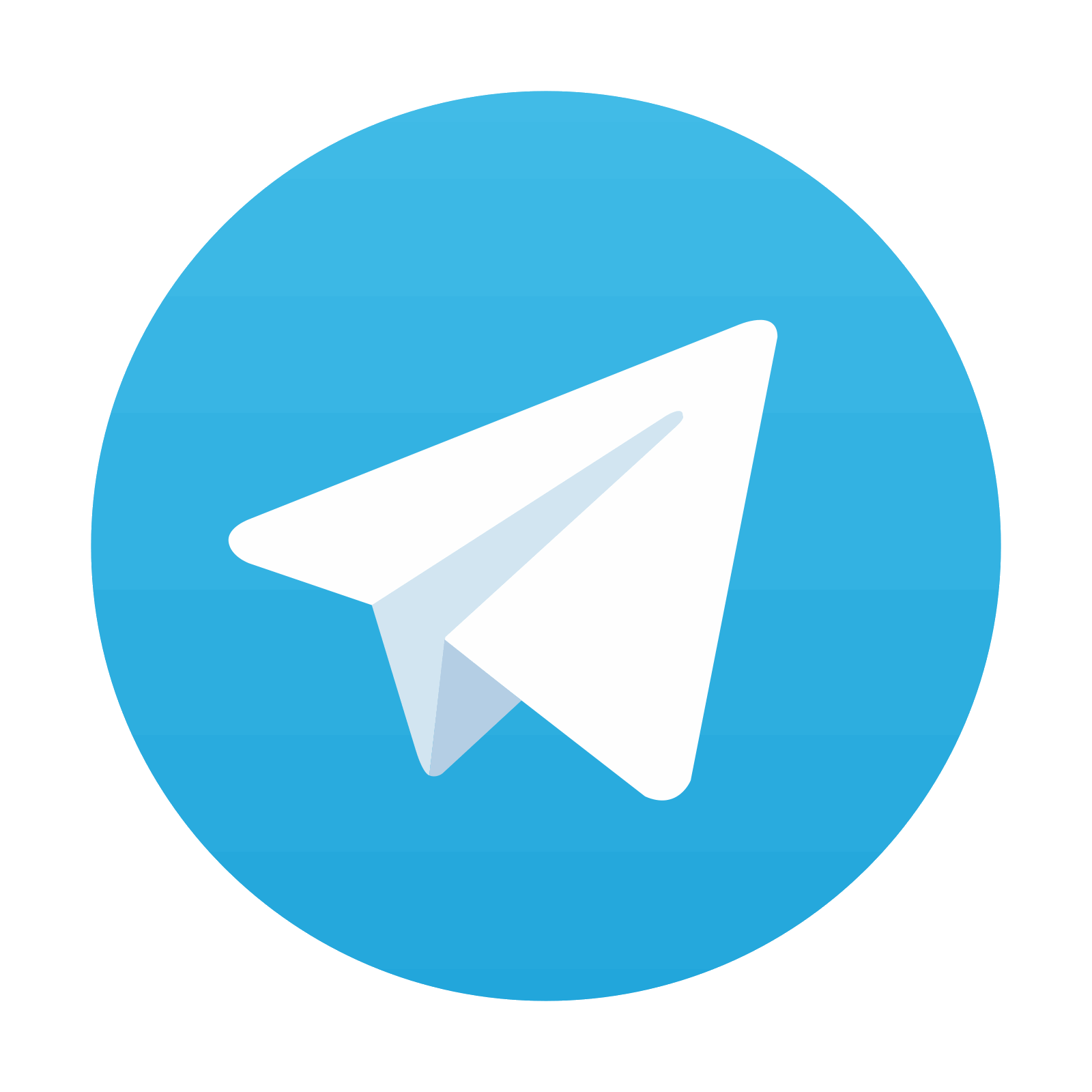
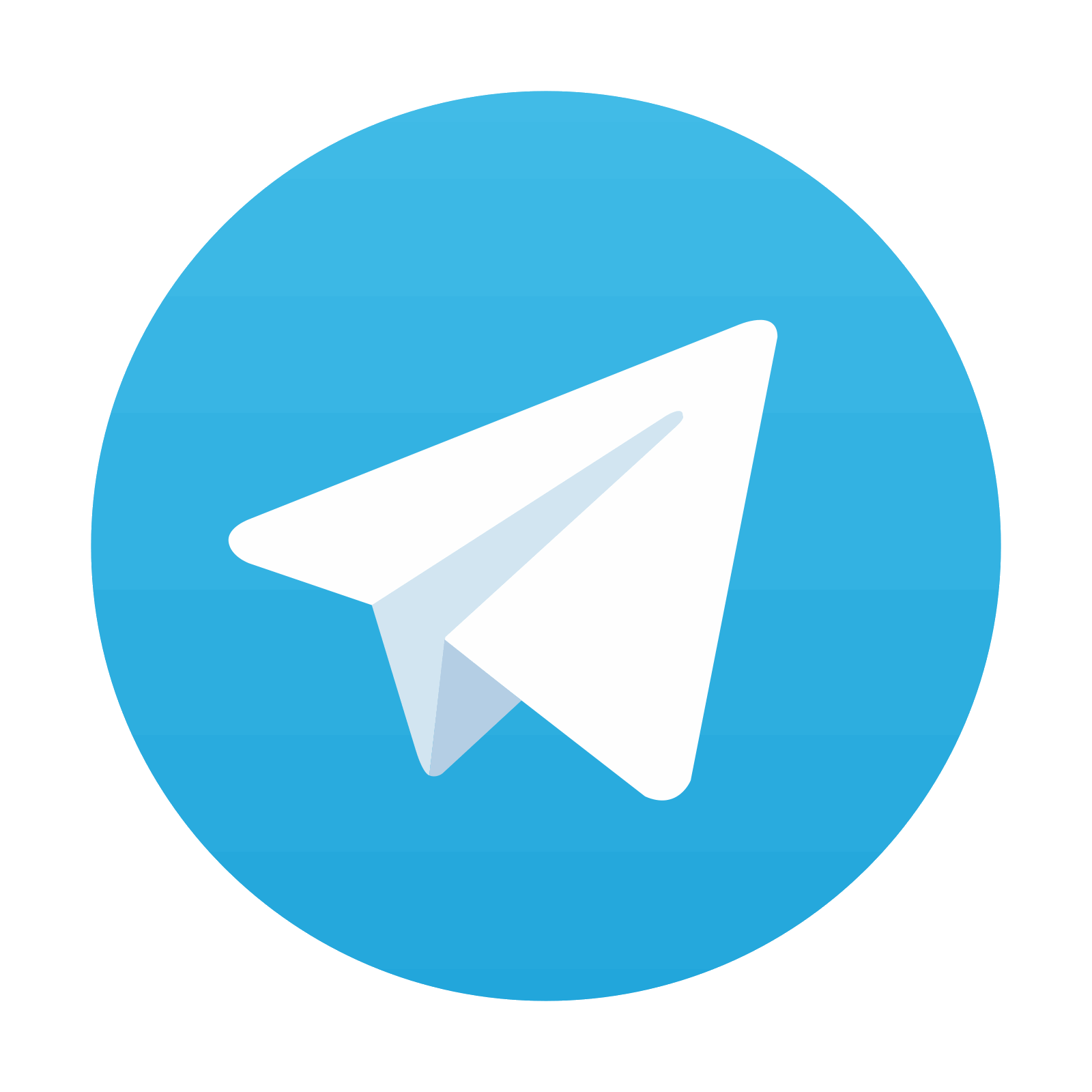
Stay updated, free articles. Join our Telegram channel
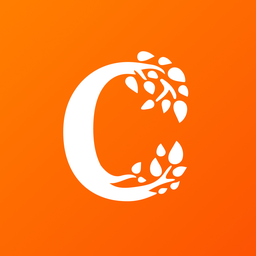
Full access? Get Clinical Tree
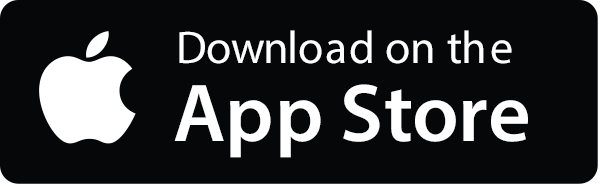
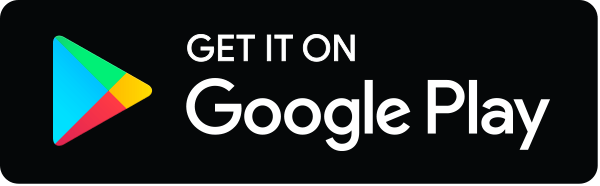
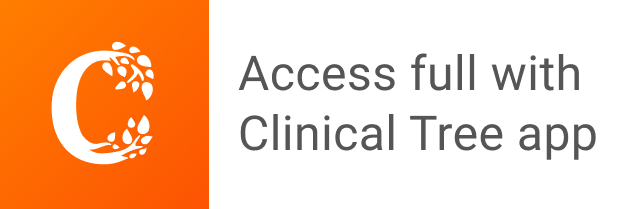