Joint Cross-talk Among Bone, Synovium, and Articular Cartilage
Courtney M. Mazur, BS
Karsyn N. Bailey, BS
Tamara Alliston, PhD
Dr. Alliston or an immediate family member is a member of a speakers’ bureau or has made paid presentations on behalf of Orthofix, Inc. Neither of the following authors nor any immediate family member has received anything of value from or has stock or stock options held in a commercial company or institution related directly or indirectly to the subject of this chapter: Ms. Mazur and Ms. Bailey.
Keywords: articular cartilage; joint cross-talk; osteoarthritis; subchondral bone; synovium
INTRODUCTION
Diarthrodial joints facilitate smooth motion and load transfer through integrated function of multiple tissues, including articular cartilage, bone, ligaments, tendons, synovium, and menisci. Under normal conditions, the biological and mechanical activities of these tissues are exquisitely coordinated. They develop together, often share a blood supply, exchange signaling molecules, and mechanically load each other almost constantly throughout life. While cross-talk among these tissues in healthy joints supports homeostasis, it can alternatively drive a positive-feedback loop of joint destruction if any of the joint components become perturbed by injury or diseases, such as osteoarthritis (OA) (Figure 1).
OA is now considered a “whole-joint” disease, in recognition of the coordinated degradation and interaction of multiple tissues within the joint. Examining OA through the lens of joint cross-talk sheds light on disease origin and progression, joint pain, and therapeutic strategies. Though evidence supports the cross-talk of muscle, ligaments, menisci, nerves, and gut microbiota with joint tissues, this chapter will focus on the interactions among bone, synovium, and articular cartilage in the most common joint disease, OA. This chapter will review evidence for cross-talk at the whole-joint level and the cellular level, relying on data from humans unless otherwise noted, discuss some possible mechanisms of cross-talk among these tissues, and conclude with a discussion of therapies in development for joint disease that attempt to harness these tissue interactions.
CLINICAL EVIDENCE OF CROSS-TALK
BONE AND CARTILAGE CROSS-TALK
BONE MARROW LESIONS
Bone marrow lesions (BMLs), also known as bone marrow edema or bone bruises, appear in injured subchondral bone and serve as markers of the pathological joint cross-talk observed in OA. BMLs are detected noninvasively by MRI, where they appear as areas of ill-defined hyperintense signal in fluid-sensitive, fat-saturated image sequences (Figure 2). In T1-weighted images, they appear as areas of low signal intensity. There is some evidence that lesions detectable by both types of sequence are more severe than those detectable by fluid-sensitive sequences only.1 The presence of BMLs is clinically important because they forecast joint pain and osteoarthritic cartilage changes.
BMLs, while primarily a feature of bone, are strongly associated with OA severity in human joints in in vivo and ex vivo studies. Relative to healthy joints, osteoarthritic knees have more BMLs, excessive trabecular bone, and a
thicker subchondral plate. The severity of BMLs in knees and hips also correlates with histologic evidence of OA severity such as scores on the OARSI Osteoarthritis Cartilage Histopathology Assessment System, tidemark duplication, and cartilage volume.2 Interestingly, BMLs are detectable in some asymptomatic patients, suggesting that they may precede other signs of OA.
thicker subchondral plate. The severity of BMLs in knees and hips also correlates with histologic evidence of OA severity such as scores on the OARSI Osteoarthritis Cartilage Histopathology Assessment System, tidemark duplication, and cartilage volume.2 Interestingly, BMLs are detectable in some asymptomatic patients, suggesting that they may precede other signs of OA.
BMLs can be monitored noninvasively over time, making them a useful clinical tool. In longitudinal imaging studies spanning 6 weeks to 30 months, individual BMLs have been observed to appear, grow, shrink, or resolve.3,4 BMLs that grew were strongly predictive of nearby cartilage loss, whereas patients with no BMLs had minimal cartilage loss. Improvement of BMLs is associated with less frequent and less severe pain.5 Therefore, it is possible that future treatments for OA could be assessed based on their ability to shrink BMLs.
Although additional research is needed to evaluate the mechanisms by which BMLs arise or resolve, current evidence suggests that both biological and mechanical factors participate in BML formation. BMLs are frequently located in the overloaded medial compartment of varus-aligned knees and in the lateral compartment of valgus-aligned knees. Altered joint loading may also render the subchondral bone more susceptible to microfractures and to infiltration of synovial fluid or vasculature. Whether BMLs causally contribute to cartilage degradation remains to be determined. Nevertheless, BMLs reveal pathologic cross-talk between cartilage and subchondral bone and may be useful for early identification of OA, especially following joint injury.
SUBCHONDRAL CHANGES IN OSTEOARTHRITIS
In addition to cartilage fibrillation and loss, osteoarthritic joints undergo a multiphasic process of subchondral remodeling, which in turn affects cartilage homeostasis. Beneath the articular cartilage lies a layer of highly mineralized calcified cartilage, followed by the subchondral plate, and finally the subchondral trabecular (or cancellous) bone. Each of these layers undergoes distinct changes in early and late-stage OA, so it is important to consider their roles separately. Better understanding of cross-talk between subchondral bone and cartilage may improve the success of efforts to regenerate or replace articular cartilage, which often overlook the concomitant changes in these underlying tissues.
Trabecular Bone Microarchitecture
The subchondral trabecular bone, which lies between the subchondral plate and the epiphyseal plate, is responsible for transmitting loads from the joint to the skeleton. Both the rods, the elongated cylindrical trabeculae, and the plates, the large flatter trabeculae, are essential for this function. In early OA, the remodeling rate in trabecular bone increases, meaning that newly deposited organic bone matrix does not have as much time to accumulate mineral before being resorbed again.6 Thus, in early OA, trabecular bone experiences a net loss of bone volume and bone mineral, and it becomes more easily deformable. In late-stage OA, a persistent imbalance in bone remodeling has led to reports of both increased and decreased subchondral trabecular bone volume. These apparently different findings may result in part from methodologic differences in timepoints, experimental systems (eg, species, in vivo or ex vivo), or imaging technologies (eg, dual-energy x-ray absorptiometry [DXA] or computed tomography [CT]) for collecting outcomes in this dynamic disease process. These distinctions must be considered carefully when comparing studies.
To better understand the association of subchondral trabecular bone changes with cartilage degradation, several imaging techniques have been used to evaluate human joints. With high-resolution peripheral quantitative CT (pQCT) scans performed ex vivo, the morphology of trabecular plates and rods can be analyzed separately. In subchondral bone, trabecular plate structures contribute to the overall stiffness of the joint more than rods. In OA subchondral bone, there is a shift in the ratio of plate to rod number beneath degenerated and intact cartilage, providing a mechanical link between trabecular microarchitecture and cartilage degradation.7 Dynamic contrast-enhanced MRI studies demonstrate that OA subchondral bone has reduced perfusion and venous outflow consistent with venous stasis.8 Reduced perfusion may cause subchondral bone hypoxia, suggesting a biological cause for changes in bone remodeling rate. Several studies collectively suggest that, in a subset of OA, cartilage degeneration results from primary defects in bone, arising from differences in genetics, mechanics, injury, or other factors.
Studies with animal models of OA strengthen the hypothesis that trabecular bone remodeling precedes changes to cartilage. Dunkin-Hartley guinea pigs spontaneously develop OA after 3 months of age, but the plate-rod ratio increases at 2 months of age.7 Strain 13 guinea pigs, on the other hand, have consistently high bone mass but do not develop OA. This suggests that a simple change in bone mass is not likely a causal driver of end-stage OA, but that a change in homeostatic maintenance of stable
trabecular bone volume and increased bone remodeling may disrupt healthy bone-cartilage cross-talk. Along these lines, studies using antiresorptives before inducing OA in animal models often show less cartilage pathology. However, mixed results overall, especially in human trials (discussed more later) suggest that there is a critical time window in which preventive therapy could be effective.
trabecular bone volume and increased bone remodeling may disrupt healthy bone-cartilage cross-talk. Along these lines, studies using antiresorptives before inducing OA in animal models often show less cartilage pathology. However, mixed results overall, especially in human trials (discussed more later) suggest that there is a critical time window in which preventive therapy could be effective.
Subchondral Plate Remodeling
The subchondral plate, the thick, corticalized layer of bone between the subchondral trabecular bone and the calcified cartilage, also undergoes dynamic remodeling in OA. In early OA, the increase in bone remodeling rate causes thinning of the subchondral plate. In late-stage OA, the subchondral plate thickens, usually in the condyle with more joint space narrowing. This strong spatial association between subchondral plate thickening and cartilage degeneration also occurs in animal models of induced OA. For example, following meniscal destabilization in mice or varus/valgus tibial angulation in rabbits, the overloaded compartment shows more severe subchondral plate thickening and cartilage lesions.6 However, insertion of a metal rod into the subchondral bone of sheep to immediately induce subchondral stiffening did not lead to cartilage deterioration even after five years.9 Therefore, as in trabecular bone, the process of subchondral plate remodeling may be more important for cross-talk with cartilage than its resultant change in thickness.
One of the hallmarks of OA is calcified cartilage advancement, during which this highly mineralized layer expands into the uncalcified cartilage layer, leaving telltale tidemark duplications. As a result, the remaining uncalcified cartilage layer becomes thinner, while the surface beneath it becomes much thicker and denser. The combination of thicker subchondral plate and expanded layer of calcified cartilage creates a zone of highly mineralized tissue in late-stage OA referred to as subchondral bone sclerosis. In addition, in the rare disease alkaptonuria and in human OA, highly mineralized subchondral plate protrusions extend into the calcified and uncalcified cartilage10 (Figure 3). The calcified cartilage helps to transform shear stresses on the cartilage into compressive and tensile forces on the whole joint, so these protrusions and changes in thickness cause stress concentrations that can contribute to cartilage degradation.
At the border of cartilage and bone, the formation of osteophytes is another hallmark of OA. Stem cells from the periosteum or synovial lining undergo endochondral ossification, effectively expanding the subchondral bone and the cartilage surface. Osteophytes can be detected radiographically before joint space narrowing and are often a source of pain and limited mobility. While osteophyte size does not predict OA progression, their removal causes joint instability, perhaps indicating that they arise to stabilize a joint in the early stages of disease.6,11 In addition to the knee and hip, osteophytes are also common in hand OA (Figure 4), which occurs twice as often in women as in men. Women are at higher risk for OA overall, but the anatomic, hormonal, and other differences causing this discrepancy are not well studied. In summary, detection of subchondral bone changes prior to gross changes in cartilage indicates that bone remodeling might causally contribute to cartilage pathology, but whether its effects are reversible remains to be determined.
Subchondral Bone Porosity
While the uncalcified articular cartilage is nonvascularized and noninnervated, human subchondral bone is perforated by channels containing blood vessels and nerves (10 to 30 µm diameter) that extend as far as the calcified cartilage. Larger pores containing fat or marrow (30 to 100 µm diameter) and nonmineralized pathways between osteocytes and chondrocytes (5 to 50 nm diameter) have also been identified. Under normal conditions, the size and density of these channels vary within the joint, and studies with fluorescein tracers show that they are functional pathways for diffusion of small molecules between the general circulation, the subchondral bone, and the uncalcified cartilage12,13 (Figure 5). In humans, it is believed that nearly 50% of the cartilage supply of glucose, oxygen, and other essential molecules originates in the subchondral bone vasculature, with the remainder diffusing from the synovial fluid. In OA, changes to subchondral plate porosity occur at each length scale and may contribute to disease progression by altering bone-cartilage cross-talk.
Early in OA, elevated bone remodeling causes widening of existing channels and plate thinning, ultimately increasing subchondral plate porosity.13 Formation of microcracks also creates new conduits for solute movement. In late disease, vascular and nerve invasion into the subchondral bone and calcified cartilage—sometimes as far as the tidemark—further increases solute delivery to the cartilage14,15 (Figure 6). In particular, widening of channels in the subchondral plate increases transportation of large molecules (up to 70 kDa) 30% to 100% over what would be possible in healthy bone.16 While therapeutic microfracture has been utilized in an attempt to deliver mesenchymal stem cells and other factors to the site of an articular cartilage lesion, increasing vascular access to articular cartilage may actually exacerbate an underlying disease process. At the same time, in two mouse models of OA, both chondrocyte-chondrocyte and chondrocyte-osteocyte diffusion was slowed, likely due to increased mineralization of the calcified cartilage or the degeneration of osteocyte canalicular channels in bone.13
In addition to pores connecting cartilage and bone, canalicular channels (500 nm diameter) form a rich network within bone connecting osteocytes to one another and to the vasculature. To maintain this network and control bone mineralization, osteocytes secrete proteases and acid to resorb their surrounding extracellular matrix and then replace it in a process called perilacunar/canalicular remodeling (PLR).17 Relative to bone from healthy cadaveric joints, osteocytes in subchondral bone from osteonecrotic (ON) and OA human joints show diminished connectivity through canaliculi, suggesting a functional defect in PLR in ON and OA.18,19 Experimental suppression of osteocyte PLR mimics the canalicular defects in human OA and
ON subchondral bone and produces signs of joint disease in mouse models. Though the mechanisms remain unknown, PLR suppression could impact cartilage through impaired solute transport through the canalicular network as well as through changing the mechanical properties of subchondral bone. Thus, osteocyte PLR is an important process for the biological and mechanical homeostasis of bone, and its suppression has meaningful consequences for cross-talk within the joint.
ON subchondral bone and produces signs of joint disease in mouse models. Though the mechanisms remain unknown, PLR suppression could impact cartilage through impaired solute transport through the canalicular network as well as through changing the mechanical properties of subchondral bone. Thus, osteocyte PLR is an important process for the biological and mechanical homeostasis of bone, and its suppression has meaningful consequences for cross-talk within the joint.
SYNOVIAL CONTRIBUTIONS TO JOINT HOMEOSTASIS
Healthy synovium protects the joint by preventing adherence of the synovial capsule to the cartilage, by producing synovial fluid, hyaluronic acid, and lubricin, and by removing metabolites and products of matrix degradation.20 Unlike cartilage, healthy synovium is highly vascularized and contains nociceptive fibers for pain reception, and thus serves as a nutrient and sensory source for the joint.21 The intima, the inner layer of synovial tissue, contains type A and type B synoviocytes. Type A synoviocytes resemble macrophages and phagocytes, and type B cells are secretory fibroblasts.20
Synovitis, much like subchondral bone remodeling, is actively involved in OA and often precedes detectable cartilage damage.20 This inflamed synovium, considered a source of pain in OA, produces granulation tissue that covers the synovial joint, also known as a pannus, which can then invade the subchondral bone (Figure 7). During inflammation, synoviocytes increase in number and size and recruit mononuclear lymphocytes and macrophages.21,22 This cellular influx,
coupled with the production of catabolic and proinflammatory mediators, such as cytokines, nitric oxide, prostaglandin E2, and neuropeptides, can propel joint deterioration. Other hallmarks of synovitis include an increase in angiogenesis and an increased production of proangiogenic vascular endothelial growth factor (VEGF) within the synovium. Each of these factors has well-established effects on other joint tissues.
coupled with the production of catabolic and proinflammatory mediators, such as cytokines, nitric oxide, prostaglandin E2, and neuropeptides, can propel joint deterioration. Other hallmarks of synovitis include an increase in angiogenesis and an increased production of proangiogenic vascular endothelial growth factor (VEGF) within the synovium. Each of these factors has well-established effects on other joint tissues.
The most prominent proinflammatory cytokines in the pathogenesis of OA are interleukin-1β (IL-1β) and tumor necrosis factor-α (TNF-α).21,22 These cytokines can directly result in cartilage destruction or trigger their own production or the production of other cytokines, thus resulting in a feedforward cascade of joint destruction. Proinflammatory mediators can also attract other immune cells, which can in turn secrete more cytokines. The acute inflammatory response can result in a phenotypic shift in chondrocytes, causing chondrocytes to produce additional cytokines and proteolytic enzymes that increase cartilage degeneration and induce further synovial inflammation.22 Additionally, molecules from degraded articular cartilage released into the synovial cavity can directly induce synovitis.
In addition to contributing to cartilage degeneration through release of catabolic cytokines and inducing pain in OA, inflamed synovium interacts directly with subchondral bone in OA by triggering macrophages within the synovium to differentiate into osteoclasts.21 As these macrophages differentiate, they cause bony erosions, one possible mechanism through which bone resorption is increased in early stages of OA.
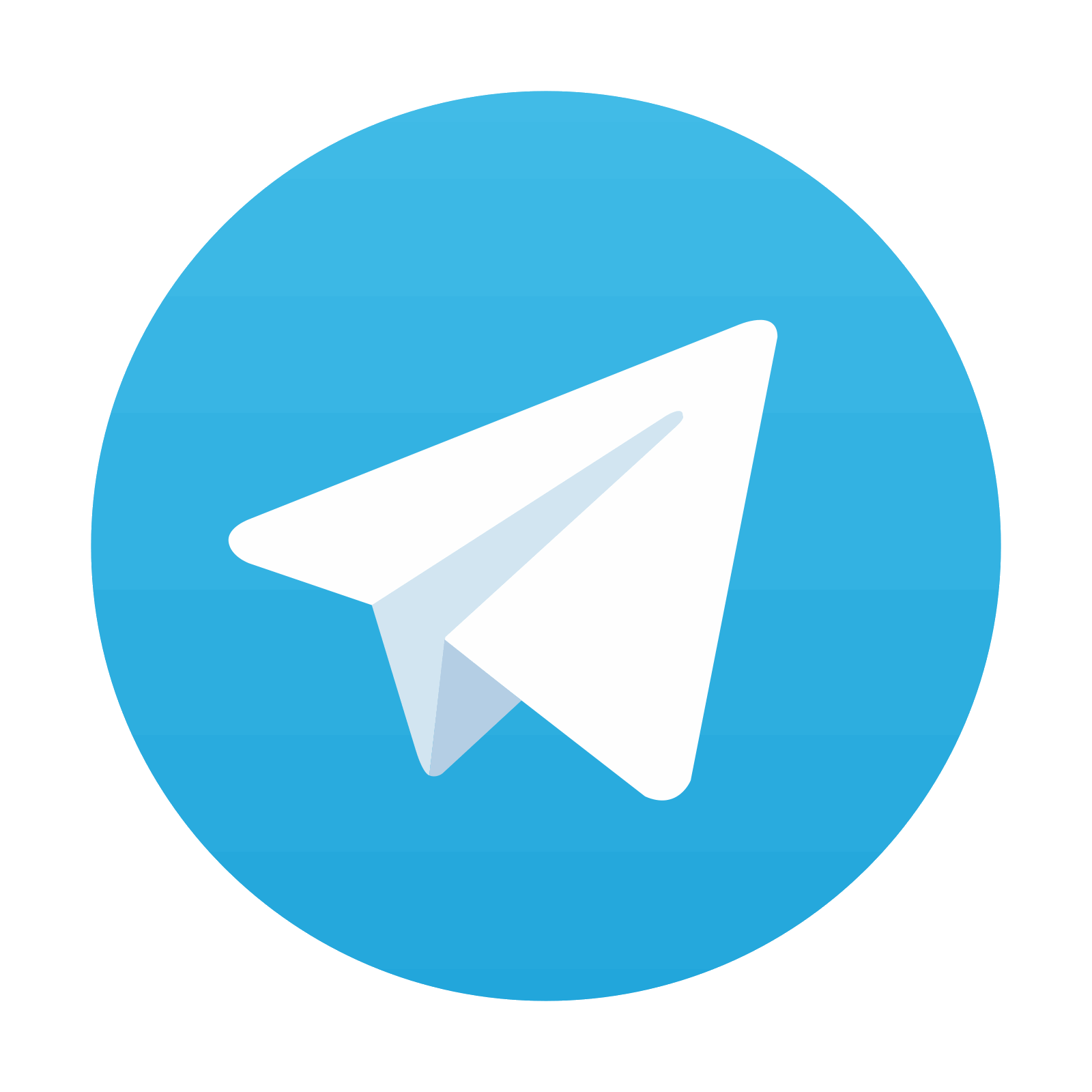
Stay updated, free articles. Join our Telegram channel
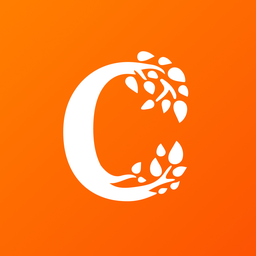
Full access? Get Clinical Tree
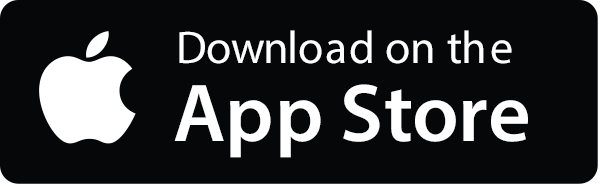
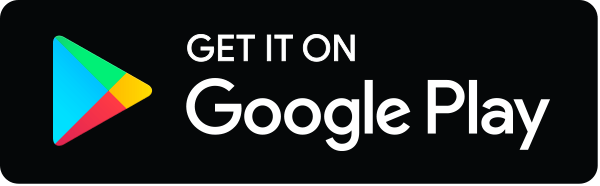