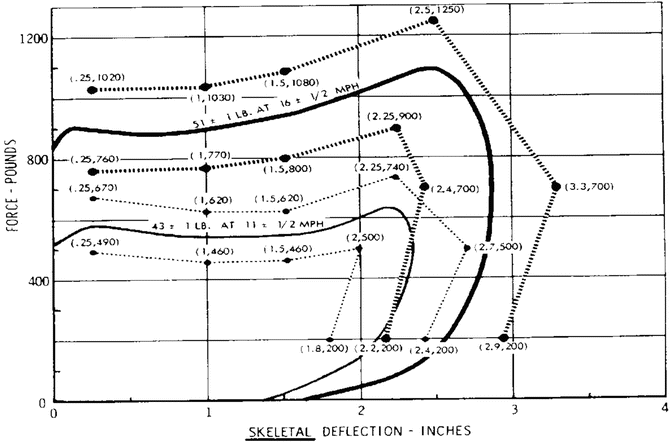
Fig. 1.2
Thoracic response to frontal impact. The two solid curves are for responses by two different impactors
To design a human surrogate (crash dummy) for impact testing, a large amount of impact response data is needed so that the dummy can be as human-like as possible. The current dummy used universally by automakers is the Hybrid III. It is shown in Fig. 1.3. Not all mechanical responses of the Hybrid III are human-like because it is very difficult to have a device that is simultaneously biofidelic and behaves in a repeatable and reproducible manner during testing. In general, the dummy is stiffer than the human and its torso is less flexible.
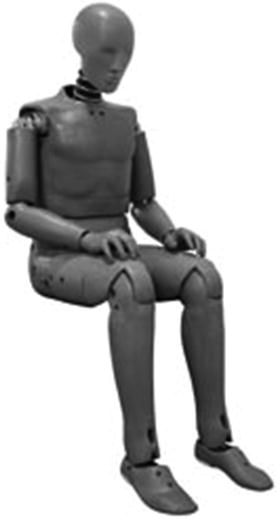
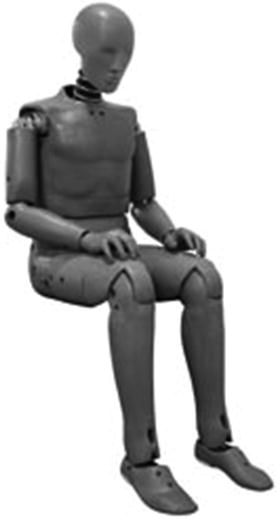
Fig. 1.3
Photograph of a Hybrid III dummy. (Courtesy of Humanetics Innovative Solutions, Inc.
1.4.3 Determination of Human Tolerance to Impact
In the design of safety systems for vehicles of any kind, it is necessary to know how much acceleration or force the body or any part of the body can take before serious injury is incurred. This is the study of human tolerance to impact. To the perennial question: How many g’s can I take? The answer is another question: How badly do you want to get hurt? There are several levels of injury and the safety design can focus on one of those levels for a given impact severity. The injury levels are:
1.
The “Ouch” level
2.
Minor injury
3.
Moderate injury
4.
Serious injury
5.
Critical injury
6.
Fatal injury
The “Ouch” level is used when testing human volunteers. If the test causes pain in any way, the test should not proceed beyond that level and the volunteer can withdraw from the program. Minor and moderate injuries are real injuries which may require a visit to the emergency room but should not require prolonged hospitalization. At the serious injury level, the healthy individual will require hospitalization but the injuries are not life threatening. They may be for the elderly and the infirm. For a vehicle to be affordable, the design should result in injuries between the moderate and the serious. Critical injuries are life threatening to the healthy individual and should be avoided. Of course, no design should result in fatal injuries.
Like response, tolerance is also highly variable among subjects and is also dependent on age and gender. Because of this variability, absolute tolerance values are not very meaningful. Instead, a probabilistic approach is taken and, for a given level of injury, the tolerance values are expressed as probability of injury. For example, for head injury at a serious injury level, the probability of injury in terms of angular acceleration is shown Fig. 1.4. In this case, the angular acceleration for 50 % probability of a minor traumatic brain injury is 5,500 rad/s2. Other parameters can be used as injury predictors, using this Logist analysis. Statistical parameters can be computed to determine which parameter is the best predictor of a particular injury.
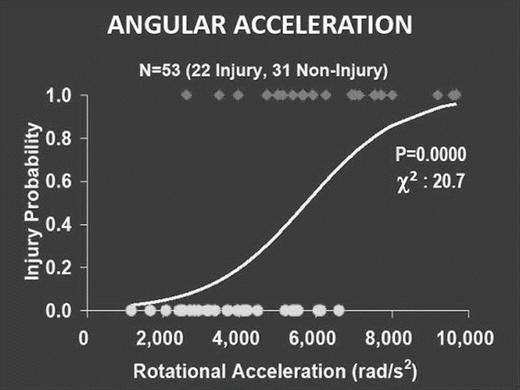
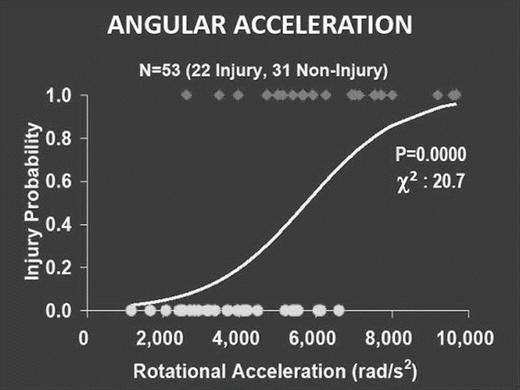
Fig. 1.4
Logist plot of mTBI versus rotational (angular) acceleration. For a 505 probability of injury the rotational acceleration is predicted to be approximately 5,500 rad/s2
1.4.4 Assessment of Safety Devices and Techniques
The knowledge gained in the three areas of study described above can be used to assess safety devices in vehicles and other systems. The two principal tools are the crash dummy (Hybrid III) and computer models. For the last half century, most of the assessment has been experimental, using the crash dummy. This assessment is made as the design is progressing and calls for many crash tests that are costly and time consuming. The automotive industry has begun to realize that computer models are now sufficiently reliable to be used as an assessment tool. With the aid of models, the designer can skip much of the crash testing and do a final test of the system at the end of the development process. There is now a consortium of model developers, in the US, funded by the automotive manufacturers to develop a single total human body model that can be used in place of the Hybrid III. This is a lofty goal that may not be achievable because all kinds of models can be developed quickly and at low cost whereas a repeatable and reproducible crash dummy takes years of work to come to fruition. Nevertheless, this modeling effort is a laudable goal that will save the industry time and money.
1.5 Methods to Study Injury Biomechanics
Impact biomechanics began as an experimental discipline, much like many other fields of study. From observation, it progressed to testing and organized experimentation. The study began with injuries to particular body region and was gradually extended to the whole body. For example, Gurdjian and Lissner [6] concentrated their efforts on head injury and performed experiments on human skulls initially before venturing to test anesthetized animals. Evans and Lissner [7] also initiated studies on the thoracolumbar spine by testing embalmed whole cadavers on a specially designed vertical accelerator that was housed in an elevator shaft of an eight-story building. Mertz and Patrick [8] studied human neck response using a horizontal sled. Later on, the response and tolerance of the knee was also studied using horizontal sleds.
Mathematical models were developed in conjunction with the experimental studies, usually after some experimental data have become available to test the viability of the model. As computers became faster and capable of storing large amounts of data, the models became more complex and more detailed. A record of model development over half a century can be found in Yang et al. [9]. It goes without saying that most researchers have more faith in experimental data than in the predictions of mathematical or computer models. As a result, it is traditional to expect the model developers to validate their models against appropriate experimental data. The policy of the Stapp Crash Journal is that every model published in the journal must be validated. As a result, improvements in model predictions have been rapid and they are becoming reliable predictors of response and injury. In fact, because of the ready availability of models of many body regions, it is now possible to model the experiment and determine what can be expected to occur in an experiment, where to make the critical measurements and what levels of force or acceleration are to be expected in a given series of experiments. For example, in a current project on blast-related brain injury, the modelers are being asked to determine where to expect the maximum pressures in the brain and what pressure levels will be reached before any testing is done. These predictions will assist with the placement of pressure transducers in the brain to measure the peak pressures.
1.6 Injury Mechanisms from Head to Toe: Application to Design
1.6.1 Head Injury Mechanisms
1.6.1.1 Brain Injury Mechanisms
The existence of two injury mechanisms for the brain is well known. Gurdjian et al. [10] proposed that linear acceleration producing pressure waves within the brain was a mechanism for brain concussion. On the other hand, Ommaya et al. [11] were of the opinion that angular acceleration was the prime cause of concussion. This debate became quite heated for a time in the 1970s but has since died down somewhat when it became apparent that both forms of acceleration usually increased in a monotonic fashion with increased impact severity. However, the injury mechanisms due to these two forms of acceleration are quite different. Based on the work of Hardy et al. [5], we can say that angular acceleration is responsible for the relative motion of the brain within the skull during a blunt impact and that the resulting diffuse axonal injury is due to this motion. Although axonal stretch has not been measured directly, it can be deduced that this relative motion of the brain is essentially the mechanism that can stretch the axons. As for linear acceleration, it causes a pressure wave to be generated, starting as a compressive wave at the site of impact and becoming a reflected tensile wave as it is reflected from the skull at the opposite or contrecoup side. Gurdjian et al. [12] invented the fluid percussion method of causing concussion in dogs after they discovered the existence of a transient pressure wave traversing the brain. Fluid percussion tests that varied in duration from less than 1 ms to 46 ms and in magnitude from 34.5 to 345 kPa (5–50 psi), resulted in concussion in experimental animals. Unfortunately, no biomarkers were identified to describe the injury. Thus, we know that pressure is an injury mechanism but we do not know what cells are injured or what parts of the cell are damaged by pressure. Ongoing research to study the effects of blast overpressure on military personnel may soon reveal one or more mechanisms of injury at the cellular and/or the molecular level. Preliminary data on rodents [13] indicate that blast overpressure is causing the glial cells to go into apoptosis with possible deleterious effects on the neurons they support and that there was no axonal injury associated with blast exposure. This finding is reasonable in that there was very little head motion during a pure (primary) blast.
1.6.1.2 Injury Mechanisms for Acute Subdural Hematoma
The accepted injury mechanism for acute subdural hematoma (ASDH) is bridging vein rupture. However, from an engineering point of view, the acute formation of a hematoma from a ruptured vein violates the principles of fluid mechanics. The mechanism proposed in this book chapter is a hypothesis with no data to support its veracity. The reader is asked to consider the logic of the hypothesis and decide if it has more merit than the accepted mechanism.
The physiopathogenesis of ASDH formation has been a subject of debate since the early thinking of an organized space between the arachnoid and dura was detailed by Key and Retzius in the late 1800s [14]. This group described the structures of the meninges and experimentally determined that substances injected into the presumed subdural space did not mix with other substances within the tissue. Early researchers believed that fluids within the alleged space could move between compartments of the brain [15]. Thus, authors of this time period believed and offered evidence that a fluid-filled space existed between the dura and arachnoid [15–19]. As Weed continued his studies, he determined that the structures were fused together in embryos, but could be separated in mature animals [19]. These early investigators injected fluids into the subdural area and visualize the distinctive compartmentalization of these fluids. Microscopically, layers of unique cells between the dura and arachnoid tissue were recognized and these cells were thought to produce a fluid which appeared to be present within the ‘space’. Leary [20] concluded that the inner dura was lined with fibroblasts and that the cells lining the outer arachnoid were dissimilar. Thus, investigators began examining the dura and arachnoid as two exclusively separate identities.
The Dura Mater. The dura mater appears to be a thick layer of fibroblasts and extracellular collagen [21]. The cells look large and flattened and the collagen is abundant and somewhat organized. Haines [14] summarized the dura-arachnoid organization. The dura is characterized as having an inner and outer portion. The periosteal dura is adherent to the inner skull and the meningeal layer of the dura, contains a specialized layer that Nabeshima et al. [22] named the dural-border cell layer. This layer appears to be continuous with the dural aspect of the arachnoid and the histological aspects of this dural-border cell layer have brought much interest to researchers [22–25]. This amorphous layer appears to have flattened cell processes, varying sizes of extracellular spaces and little collagenous material. The amorphous structure possibly makes this an area of weakness within the tissue. A cross-section of the meninges and cell layers is shown in Fig. 1.5. If an ASDH is to form, the bleed needs to occur in the border cell layers.
The Arachnoids. The arachnoid portion of the meninges also consists of two distinct areas, the arachnoid barrier cell layer, which is attached to the dural-border cell layer, and the arachnoid trabeculae, which is closely attached to the pia mater. Both the cells and the extracellular material are dissimilar as compared to the dura mater. The cells are larger, more densely packed, having numerous mitochondria and filaments within their cytoplasm making the layer distinctive [22, 23, 26]. This closely packed structure of the arachnoid barrier cell layer excludes the presence of extracellular space, making it distinctive from the attached dural-border cell layer. Existing literature supports this idea. The description of the layers above has been verified [14, 27, 28] and testing has shown that the ‘space’ is not pre-existing. However, the junction between the dural and arachnoid border cells would be an area of weakness in cases of brain impact injury because the loosely organized dural border cell layer is attached to the more rigid arachnoid barrier cell layer. In fact, there is evidence that the space is easily created by a mechanical separation [29–31]. Since the biomechanical properties of the border cell layers have not been investigated, the adhesive properties of the layers in radial traction or in shear need to be quantified. These properties are crucial to the understanding of the formation of ASDH because of the close association of the bridging vein and cortical arteries within these layers. Only when this mechanism is established will preventative and clinical strategies be able to be discovered and tested. This will ultimately decrease morbidity and mortality rates associated with these types of brain injuries.
On the other hand, neurosurgeons are often of the opinion that the dura is attached to the skull and the arachnoid goes with the brain. Thus, even if there is no space in the subdural layer in the young, an actual space maybe created in the elderly should their brain shrink because not all of that space can be accommodated in the CSF layer. Since this is still controversial, we need to consider the mechanism of ASDH with no subdural space as well as in the presence of a subdural space occupied by CSF.
Anatomy of Cortical Vessels. The cortical vessels consist of bridging veins and cortical arteries and veins. The bridging veins traverse the dural/arachnoid complex. Their rupture has been traditionally considered responsible for ASDH and they have been studied extensively by researchers [32, 33]. The number of veins and their range of diameters have all been documented. Yamashima and Friede [34] provided a detailed description of the vessel wall as it traverses the dura/arachnoid complex in a straight course with no tortuosity to allow for the possible displacement of the brain. The cranial end is firmly attached to the rigid dura while the cerebral end is attached to the movable hemisphere. Leary [20] found that the thickness of the bridging vein walls varied remarkably in the subdural portion, the thinnest part measuring 10 μm with a range of 10–600 μm. In the subarachnoid portion, the walls have a more consistent thickness of 50–200 μm. The collagen fibers in the subdural portion were loosely woven with a pattern that was more resistant to distension while less resistant to traction. That is, bridging veins are vulnerable to leakage in the subdural region. In fact, Yamashima and Friede [34] speculated that the bridging vein can rupture in the dura/arachnoid complex due to a physiological increase in venous pressure or due to cardiac resuscitation as well as due to a head impact. Trotter [35] regarded the rupture of the bridging vein as the cause of chronic subdural hematoma. However, another bleed source is the cortical artery traversing the dura/arachnoid complex. Information on the size, distribution and number of cortical vessels is sparse. Cortical arteries are found in the CSF layer. They run along the surface for a short distance and penetrate the pia to enter the cerebral cortex. However, some of the arteries running under the arachnoid can extend branches into the subdural layer. There is even evidence of a cortical artery forming a kink (knuckle) in the subdural space, as shown in Fig. 1.6 [36]. When the dura separates from the arachnoid, the vessel wall of the knuckle is torn off and bleeding from this tear results in an ASDH.
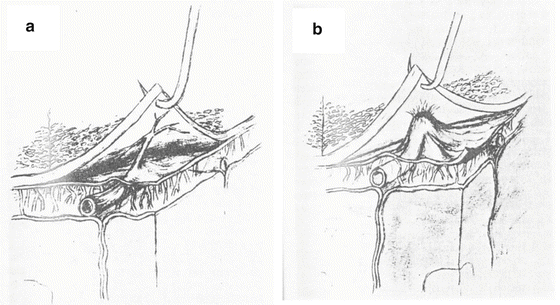
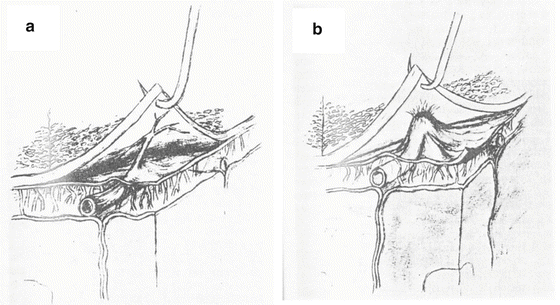
Fig. 1.6
(a) Bridging cortical artery connected to the dura. (b) Adherence of cortical arterial knuckle to dura and arachnoid (Bongioanni et al. [36])
Acute Subdural Hematomas. Subdural hematoma (SDH) is a clinical condition due to a quickly clotting blood collection amid the dura and arachnoid membranes. ASDH’s are most frequently the result of an acute head injury, however they can sometimes occur spontaneously in the elderly. The mechanism behind the separation of the arachnoid from the dura has yet to be determined. ASDH’s usually transpire when the brain is subjected to a high energy, short duration force from trauma. It is thought that this shearing force will tear the bridging veins and as a consequence ASDH will form. However, epidemiological studies have shown that injuries other than bridging vein rupture accounted for a significant portion of ASDH cases. Thus, the need to determine the mechanism behind the injury is vital before any effective preventative and therapeutic strategies can be attempted and implemented. Finding the pathogenic mechanism through a more open-minded approach will lead to new innovative treatments for this disabling condition.
Epidemiology. Traumatic ASDH’s are among the most lethal of all head injuries, carrying the highest risk to the patient, with a mortality rate of greater than 50 % in most studies. ASDH kills or severely disables more head injured patients than any other complication of cranial trauma. The main pathological factor involved is ischemic neuronal damage that results from cerebral vascular damage, raising the intracranial pressure. ASDH was found in patients who were involved in motor vehicle crashes, falls and assaults [37]. It is also found in boxers [38]. According to Gennarelli and Thibault [39], ASDH is the most important cause of death in severely head injured patients due to high incidence (30 %), high mortality (60 %) and head injury severity (2/3 with Glasgow Coma scores of 3–5). They also found that the cause of ASDH by falls or assaults was 72 % while that due to motor vehicle crashes was only 24 %. Maxeiner [40] attributed the source to bleeding in ASDH cases to extensive brain surface damage (contusion) and ruptured superficial cerebral vessels, including bridging veins and small arteries of the cortex. However, he also indicated in another publication [41] that rupture of the bridging veins did not lead to the formation of ASDH’s. In fact, Maxeiner and Wolff [42] showed that there was an equal probability of ASDH caused by bridging vein rupture and by cortical artery rupture. Moreover, Shenkin [43] reviewed 39 consecutive cases of ASDH and found that there was a high incidence of cortical artery rupture (61.5 %). Bleeds of venous origin constituted 25.6 % of the cases and cerebral contusions were the cause in 7.7 % of the cases. The elderly were found to be more susceptible to ASDH [44, 45]. Since there can be brain shrinkage with age resulting in stretching of the bridging veins, the high incidence among the elderly can be explained by bridging vein rupture. However, the simple rupture of the bridging vein should not lead to ASDH formation unless additional mechanical factors are present, as explained below. Thus, clear mechanisms of ASDH formation need to be formulated before we can claim to understand why there is a high incidence of ASDH among the elderly. Karnath [46] found that ASDH usually occurs in younger adults while chronic SDH usually occurs in older individuals between 60 and 70 years of age. Finally, although the literature is silent in terms of a detailed injury mechanism, there is an implication that ASDH occurs when there is head contact with a rigid surface. However, in their experiments on subhuman primates, Gennarelli and Thibault [39] applied a pure angular acceleration to the head without direct impact to cause ASDH in these animals. More than one injury mechanism is in play in the formation of ASDH.
In terms of the locations of ASDH, not all ASDH’s occur along the superior sagittal sinus into which the bridging vein empties the venous blood. Obviously, non-bridging vein related ASDH’s are caused by bleeds from other sources, such as cortical vessels and brain contusion or laceration [47]. We will now consider the mechanism of ASDH formation from cortical bleeds for reasons stated in the section below.
Biomechanical Mechanisms for the Formation of ASDH
Based on current thinking, ASDH can arise from one of three sources, the first being the cortical arteries and veins. Laceration or rupture of these vessels can occur with penetrating injuries. Secondly, closed head injuries resulting in large contusions can cause similar bleeding into the adjacent subdural area. Thirdly and the most common type of ASDH is thought to occur from tearing of the veins that bridge the subdural area as they travel from the surface of the brain to the various dural sinuses. This last mechanism assumes rupture of the bridging vein in the subdural space since if it ruptured below the arachnoid, the result would be a subarachnoid hematoma. Ultimate strain to failure of the bridging veins and possibly other tissue components is inversely related to the strain rate [48]. Thus, the threshold for injury decreases as the strain rate or acceleration is increased. Gennarelli and Thibault [39] opined that ASDH is due to the rupture bridging veins during angular acceleration of the head associated with rapid onset rates (high strain rate). They contend that nothing needs to strike the head in order for ASDH to occur. That is, although impact to the head is certainly the most common cause of ASDH, it is the angular acceleration induced by the impact and not the head contact that causes ASDH. Examples include violent non-cranial impacts of football players and motorcycle riders. A rapid head movement is sufficient to exceed the bridging vein tolerance. This group also demonstrated that sensitivity of the ultimate strain of bridging veins to strain rate and provided acceleration tolerance data for subdural hematoma in primates [39]. Lee and Haut [49] reported the insensitivity of tensile failure properties of human cerebral bridging veins to strain rate. However, data scattering drew concerns regarding this finding. To confirm their data, this group performed similar experiments on the carotid arteries and jugular veins of ferrets [50]. These vessels were stretched longitudinally in vitro at either a low (0.2–2.0 s−1) or high rate (200 s−1). The ultimate stretch ratios and loads were found to be independent of strain rate in all the vessels tested. Therefore, the results appeared to support their previous finding on human bridging veins. Maxeiner [40] tested the hypothesis that subdural hematomas are less frequent in acceleration injuries in traffic accidents compared to falls or assaults. They reported that this hypothesis did not hold true in the same way for bridging vein ruptures. Ruptures of these vessels without subdural bleeding were only seldom mentioned in the literature. However, if no subdural hematomas were present, no one would look for these structures. They predicted that the frequency of bridging vein lesions in severe head injuries was likely underestimated in the clinical as well as in the postmortem literature, hypothesizing that a rapid increase of intracranial pressure after the accident produced a collapse of the cerebral circulation which is probably responsible for the absence of the subdural hematomas in the presented cases. Most recently, Monson et al. [51] examined the mechanical behavior of human cerebral blood vessels. This group determined that cerebral arteries were noticeably stiffer than the cerebral veins. Pang et al. [52] studied the morphological properties of pig cerebral bridging vein. They demonstrated that there is a narrow cuff at the junction of the cerebral bridging veins and the superior sagittal sinus. This finding could play an important role in maintaining intracranial pressure (ICP) and ASDH formation. Collectively, all these reports implicate that failure of the bridging vein structure is associated with a large number of subdural hematomas.
However, how the failure of bridging veins causes the venous blood to form an ASDH is difficult to explain based on the principles of fluid mechanics. Since the path to the sagittal sinus is still open and the creation of a subdural space constitutes resistance to fluid flow, it is not clear how this can happen in the absence of other mechanical factors. This principle is demonstrated in Fig. 1.7. We see that for the blood to form a space, and thus an ASDH, in the border cell layer, it must overcome the adhesive resistance between the dural and arachnoid border cells and enlarge the space until it becomes symptomatic and visible on scans. On the other hand, the flow of venous blood into the superior sagittal sinus is the path of least resistance because there is no need to push apart solid boundaries (cell layers) and the formation of an ASDH in the subdural layer by venous blood would violate this very basic principle of fluid mechanics – namely, flow will proceed in the direction of least resistance.
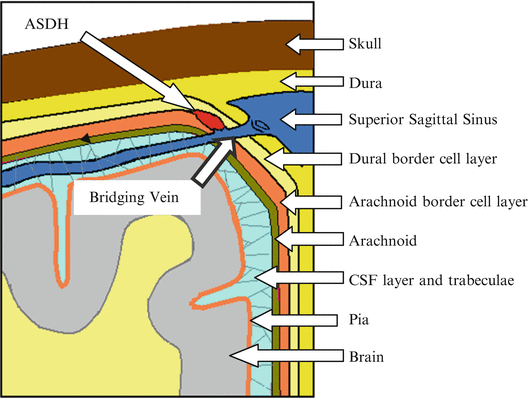
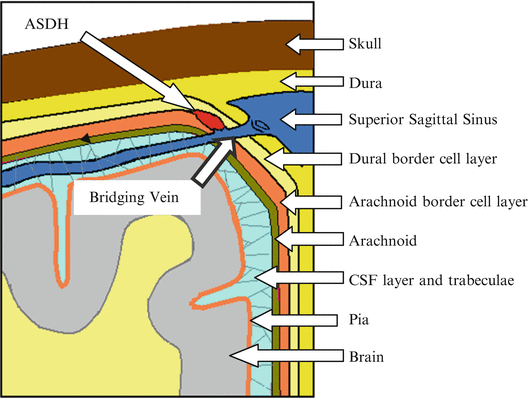
Fig. 1.7
ASDH formation due to bridging vein rupture is not possible in the subdural layer, based on principles of fluid mechanics
If we consider the possible pre-existence of a subdural space filled with CSF, then a rupture of the bridging vein in the subdural space will allow the blood to enter this space. However, it is not likely that this flow is strong enough to progress into an ASDH because the ICP in the CSF is essentially the same as the pressure in the veins.
To summarize findings to date, ASDH is found in victims of falls, assaults and motor vehicle crashes who sustain a direct impact to the head against a rigid surface. The head undergoes both linear and angular acceleration. However, Gennarelli and Thibault [39] have demonstrated the formation of ASDH with extremely high pure angular acceleration in subhuman primates and found ruptured bridging veins in these animals. Similarly, Depreitere et al. [53] used a reverse injection method to demonstrate the feasibility of ASDH formation after a direct impact that resulted in high angular accelerations. They may have indeed created the observed SDH by reverse injection which is unnatural and not representative of a venous overpressure. Nevertheless, the circumstantial evidence of associating bridging vein rupture with ASDH is extremely strong. However, as mentioned above, why would the normal flow of blood into the superior sagittal sinus be diverted into the subdural space to form an ASDH against a much higher resistance or against the ICP in the CSF? It violates the basic principle of fluid flow in that the flow will go in the direction of least resistance. We can hypothesize a series of mechanisms that can explain the observed phenomenon and provide a logical explanation for the formation of ASDH. However, before we list the various hypotheses, it is necessary to reiterate the assumption that the bridging vein rupture we are concerned with needs to occur in the dural/arachnoid complex because if it occurred below the arachnoid, a subarachnoid hematoma (SAH) would be the result. It is also necessary to reiterate the finding that the dural and arachnoid border cells can be easily separated although the amount of force needed to achieve this separation has never been measured. It is also interesting to note that a subarachnoid hematoma will form in the CSF space but a SDH will not form in the subdural space from a ruptured bridging vein. The explanation is rather simple. A torn bridging vein below the arachnoid should open the CSF to the sagittal superior sinus and there will be leakage of CSF into the sinus. As a result, venous blood from the torn bridging vein will fill the CSF space to maintain the volume of the CSF layer. Thus, a subarachnoid hematoma can easily form when the bridging vein is ruptured below the arachnoid.
Hypothesis I – Almost all ASDH’s from non-penetrating impacts are due to the rupture of cortical arteries in the dural/arachnoid complex, not including the bridging veins.
We postulate that border cell layers need to either separate radially or deform in shear to a sufficient extent to tear these cortical vessels and that the tearing of the cortical arteries will generate enough pressure in the subdural layer to cause the separation to progress, resulting in an ASDH. If this hypothesis can be validated, it can provide a logical explanation for the formation of ASDH.
Hypothesis II – Radial separation of the border cell layers can occur when there is skull in-bending and skull rebound due to a direct impact.
Not all direct impacts produce high angular accelerations but they are more likely to cause skull deformation in the form of in-bending and subsequent rebound. This in-bending can cause delamination of the border cell layer sandwiched between two layers with dissimilar material properties and the rebound applies tension to the dura to cause separation of the border cells and rupture of the cortical vessels within the dural/arachnoid complex. Large deformations can occur in the temporal area of the adult skull as well as in the skulls of children. This mechanism can also explain the formation of ASDH at sites remote from the site of impact because the skull is a closed container which will increase in diameter in one direction when the orthogonal diameter is decreased by impact. This hypothesis can be tested using an animal model as well as a computer model.
Hypothesis III – Shearing deformation of the border cell layer can rupture the cortical vessels when the head is subjected to high levels of angular acceleration.
Results of the brain motion mapping study reported by Hardy et al. [5] reveal that most of the relative motion of the brain with respect to the skull occurs near the center of the brain, above the brain stem. Relative motion of the brain surface is small but it is not non-existent under angular accelerations below 10,000 rad/s2. It is conceivable that under very high angular accelerations, the border cell layers will undergo shear deformation that is large enough to rupture the cortical vessels. We also postulate that the relative motion does not occur in the cerebral spinal fluid (CSF) layer because the trabeculae in the CSF layer have a higher shear resistance as compared to the border cells. A study to measure the shear resistance of the pia-arachnoid junction was completed by Jin et al. [54]. This hypothesis can again be tested using either an animal model or a computer model.
Hypothesis IV – Even though the bridging vein in the border cell layer may be ruptured, it does not result in the formation of ASDH.
The prevailing understanding of ASDH formation due to bridging vein rupture is inconsistent with all known principles of fluid mechanics. Yet, bridging vein rupture was found in patients with ASDH, in cadaveric subjects and animal subjects. This hypothesis can be tested by rupturing the bridging vein of an animal in the border cell layer with no head impact to demonstrate that the rupture will not cause an ASDH to form and progress. The reverse injection method used by Depreitere et al. [53] forces the contrast medium out of the ruptured vessel into the border cell layer which can then be easily separated. There is no reversal of flow of this type in vivo unless there is a large increase in venous pressure. It is also necessary to test the amount of pressure needed to reverse the flow and cause an ASDH to form. However, for an ASDH to progress, a sustained venous over pressure is needed for a period of several hours, which is physiologically unlikely to occur. If the hypothesis is valid, the inevitable conclusion is that many of the observed bridging vein ruptures were due to the autopsy or surgical procedure used to identify the ASDH or that there is cortical bleeding associated with the observed rupture that was not detected. In addition, SDH associated with a pure bridging vein rupture will likely result in a chronic SDH, from occasional increases in venous pressure, such as in a valsalva maneuver.
The reader is encouraged to do research in this area to confirm these hypotheses or to prove them wrong.
1.6.2 Neck Injury Mechanisms
1.6.2.1 The Cause of Neck Pain After Whiplash
Neck pain, resulting from being the victim of a low speed rearend impact (whiplash), is the most commonly reported injury or syndrome [55–57]. The societal cost of this syndrome has been found to be high in North America and in Europe [58–60]. Some researchers have expressed the opinion that complaints of pain and disability are related to the amount of compensation a victim can expect to receive. In Lithuania, Schrader et al. [61] found that whiplash victims all returned to work 2 weeks after the event because there is no compensation for injury. This statistic is cited as a basis for this opinion. Ferrari and Russell [62] deny the severity of the problem while Radanov [63] and Bogduk [64] are of the opinion that chronic symptoms are real and are present in 10–20 % of Bogduk’s patients 6 months after whiplash.
In terms of prevention of neck pain following whiplash, the current headrest does not seem to be effective, as evidenced by the continuing interest in the subject in the medical literature. From the biomechanical point of view, an injury cannot be prevented unless the causes or mechanisms are known. For whiplash, several hypotheses have been proposed and until the biomechanics community can come to an agreement as to the true cause of whiplash, prevention of the syndrome would be difficult. The discussion on neck pain and whiplash hypotheses provided below is taken from Deng et al. [65].
To understand the neck pain incurred due to rear-end impacts, current knowledge about spinal pain is reviewed first. The brain interprets acute pain from the signals it receives from a network of pain-sensing nerve endings or nociceptors that are found throughout the body. They are quite numerous in the skin, muscles and tendons but are not normally found in articular cartilage of synovial joints. Nociceptors have a high threshold. That is, a large stimulus is needed to get them to fire and to signal the brain that there is potential or real hazard to that region of the body. This means that the tissue in which the nociceptor is imbedded needs to be deformed considerably before the nociceptor is set off. It is also known that when the soft tissue in the intervertebral joint is inflamed due to degenerative processes, the threshold of nociceptors is reduced and much less deformation is necessary to cause pain.
In normal intervertebral discs, nociceptors are rather sparse and focused on the peripheral regions. They may not even be sensitive to mechanical loading. However, in a degenerated disc, nociceptors become more numerous and are sensitive to mechanical loading. Elsewhere in the intervertebral joint, nociceptors are found in the facet capsules, spinal ligaments, tendons and muscles. In the lumbar spine facet capsule, Cavanaugh et al. [66] were able to show that facet capsules are a source of low back pain. Bogduk and Marsland [67] and Barnsley et al. [68] have clinical evidence of cervical facet pain after whiplash.
There is also another type of pain related to the spine. It is a radicular or radiating type of pain felt in the extremities as a result of degeneration in the intervertebral joint. It is well known that numbness and tingling in the arms are due to pressure on the nerve roots exiting the spinal cord. The pressure can be from a bulging or herniated disc or from bony overgrowth narrowing the foramen through which the nerve exits. The reason for radicular pain is still not entirely clear but Ozaktay et al. [69] were able to show that a toxic chemical found in the nucleus pulposus can inflame the nerve root and produce this referred or phantom pain. If the dorsal root ganglion is affected by this chemical, the pain can be quite severe. However, it is important to recognize that mechanical or chemical effects on the nerve root cause radiculopathy or pain that appears to be located in the extremities and not localized pain in the area of the intervertebral joint. There are at least five different hypotheses, which offer explanations for the source of neck pain following whiplash. These hypotheses are reviewed briefly below.
MacNab [70] proposed an early hypothesis, identifying neck hyperextension as the injury mechanism. This was based on primate studies in which the headrest was not used and head and neck extensions exceeded 90°. However, current automotive head restraints were not effective in reducing the frequency of this problem. As a result, other hypotheses were proposed.
A popular theory is muscle injury from hyperextension, during which the anterior cervical muscles undergo an eccentric contraction. That is, the muscles are contracting as they are being stretched. It has been shown by Garrett et al. [71] that muscles can only be injured during eccentric contraction. However, in the case of these anterior muscles, they tend to hurt for few days after the impact but are not a chronic problem. The difficulty appears to be located in the back of the neck where the extensor muscles are located. These muscles undergo concentric contraction and are not likely to be injured. Tencer et al. [72] proposed that the injury to the extensor muscles occurred during rebound of the head and neck as they undergo eccentric contraction. The mechanism is biomechanically consistent with the results of Garrett et al. [71] but the hypothesis flies in the face of many more frontal impacts in which there is hyperflexion due to severe crashes and there are not a large number of complaints of neck ache from these crash victims. More recent data by Azar et al. [73, 74] show that stretching of the facet joint capsule can induce muscle spasms and pain. That is, muscular involvement is secondary to facet capsular stretch.
Ono et al. [75] and Yoganandan et al. [76] both proposed a facet joint impingement injury mechanism. Specifically, Ono et al. [75] theorized that a portion of the facet capsule can be trapped between the facet joint surfaces and pinched, causing pain. There is no biomechanical evidence that the capsule is loose enough to be trapped between the facet joint and even it was trapped, evidence is lacking to show that nociceptors are present in the trapped portion of the capsule and are indeed set off by the pressure. The proposition that compression of the facet surfaces can produce pain is also untenable since cartilage is devoid of nociceptors and there is no neurophysiological evidence that the nociceptors in the subchondral bone can be made to fire by this presumed compression.
Aldman [77] found that there was a pressure increase in the spinal canal during whiplash causing pressure on the nerve roots. Subsequent studies by Svensson et al. [78] revealed that this pressure can affect the dorsal root ganglion (DRG) causing it to send pain signals to the brain. Stimulation of or pressure on the nerve roots and DRG will produce radicular pain and not pain in the neck. Furthermore, a generalized increase in spinal canal pressure cannot selectively affect the nerve roots and DRG in the lower cervical spine where most of the problems appear to reside. The originators of this hypothesis also failed to provide objective evidence regarding DRG dysfunction due to transient pressures. Because of these inconsistencies, this hypothesis is not likely to be valid. While political support is keeping this hypothesis viable it has very little scientific merit.
Yang and Begeman [79] proposed the shear hypothesis which attributes the pain to the facet capsule which can be stretched during whiplash. The motion of the torso precedes that of the neck and in order for the head to remain attached to the torso, a shear force is generated at each cervical level and transmitted up the cervical spine until it reaches the occipital condyles where the force can act on the head to cause it to move forward. This shearing action causes relative motion between adjacent vertebrae which can be most pronounced at the lower cervical levels where the facet angle is less steep. It remains to be shown that facet stretch is large at these lower cervical levels and that there is indeed sufficient stretch to cause the nociceptors to fire and thus cause pain.
In support of the shear hypothesis, neurophysiological studies were performed by Lu et al. [80] to show that nociceptors in the goat facet joint capsule could be made to fire if the capsule was stretched both statically and dynamically. The capsular strains reached were found to be as high as 60 %. These strains are consistent with the cadaveric studies by Deng et al. [65] who found large capsular strains as high as 92 % and who also found relative sliding and rotation between adjacent vertebrae, confirming the shear hypothesis.
With the aid of active safety technology, we now can eliminate the whiplash problem by designing the seat back and headrest to work together during a rear impact. The idea is to eliminate shear forces in the neck. We will need a collision sensor on the rear bumper to provide the signal for an impending rearend collision. This signal will activate the headrest and bring it forward to contact the head of the occupant prior to collision. During the collision, the head and torso need to be moved forward at the same rate to eliminate shear between the cervical vertebrae. It is likely that such a seat is already available but it has not been fine tuned to accomplish the zero shear objective. The stiffnesses of the headrest and seat back need to be selected to match those of the head and torso so as to minimize relative motion between the head and torso. If this last step can be accomplished, we will have a seat that can prevent whiplash associated disorders.
The conclusion is that unless and until the biomechanics community accepts a single hypothesis for whiplash related pain, no effective preventive measure can be implemented, even though a solution is at hand.
1.6.3 Injury Mechanisms of the Thorax
1.6.3.1 Traumatic Rupture of the Aorta
Of all the traumatic injuries to the thorax, traumatic rupture of the aorta (TRA) due to blunt impact has the highest fatality rate, even among victims who made it to the hospital. When a frank rupture occurs at the crash scene, it is very difficult to save the victim. TRA related to automobile crashes has been known to auto safety professionals and surgeons for a long time [81]. The injury was originally found to be due to chest impact with the steering wheel at barrier impact speeds in excess of 45 mph. Recently however, TRA was found to occur in side impact after belt and airbag restraints were introduced to mitigate the effects of a frontal collision. One Canadian statistic indicates that the fatality rate due to TRA in the early 1990s was 21 % of all lateral impact cases.
One of the first known attempts at reproducing TRA experimentally was the work of Roberts et al. [82] who impacted anesthetized dogs in the sternum and achieved aortic rupture. A flash x-ray system was used to track the movement of the aorta but it is not clear how many ruptures were obtained out of the six animals they impacted. It can be confirmed that the rupture was transverse. Subsequent efforts to reproduce this injury in cadavers were largely unsuccessful [83] until Hardy et al. [84] managed to cause TRA in seven of the eight cadavers tested. They were tested in an inverted position with the lower extremities removed and were held up for pendulum impact by a clamp that went around the vertebral column. The peri-isthmus region of the aorta was targeted with radio-opaque markers and was visualized during impact by means of a biplanar high speed x-ray unit. Video images of aortic motion and deformation were recorded at 1,000 frames per second. The impact speeds varied from 6.8 to 12.2 m/s and the impact modes took form of shoveling, side impact, submarining and combined. These modes are described in detail [84] and they all were able cause TRA in the cadaver. Most of the tears were transverse with two oblique tears. The failure of the aorta was tensile in nature and the failure strains were estimated to vary from 4.1 % to 35.6 % near the site of failure. It was concluded that the failure mechanism was tension in the aorta that developed when it moved away from the impactor.
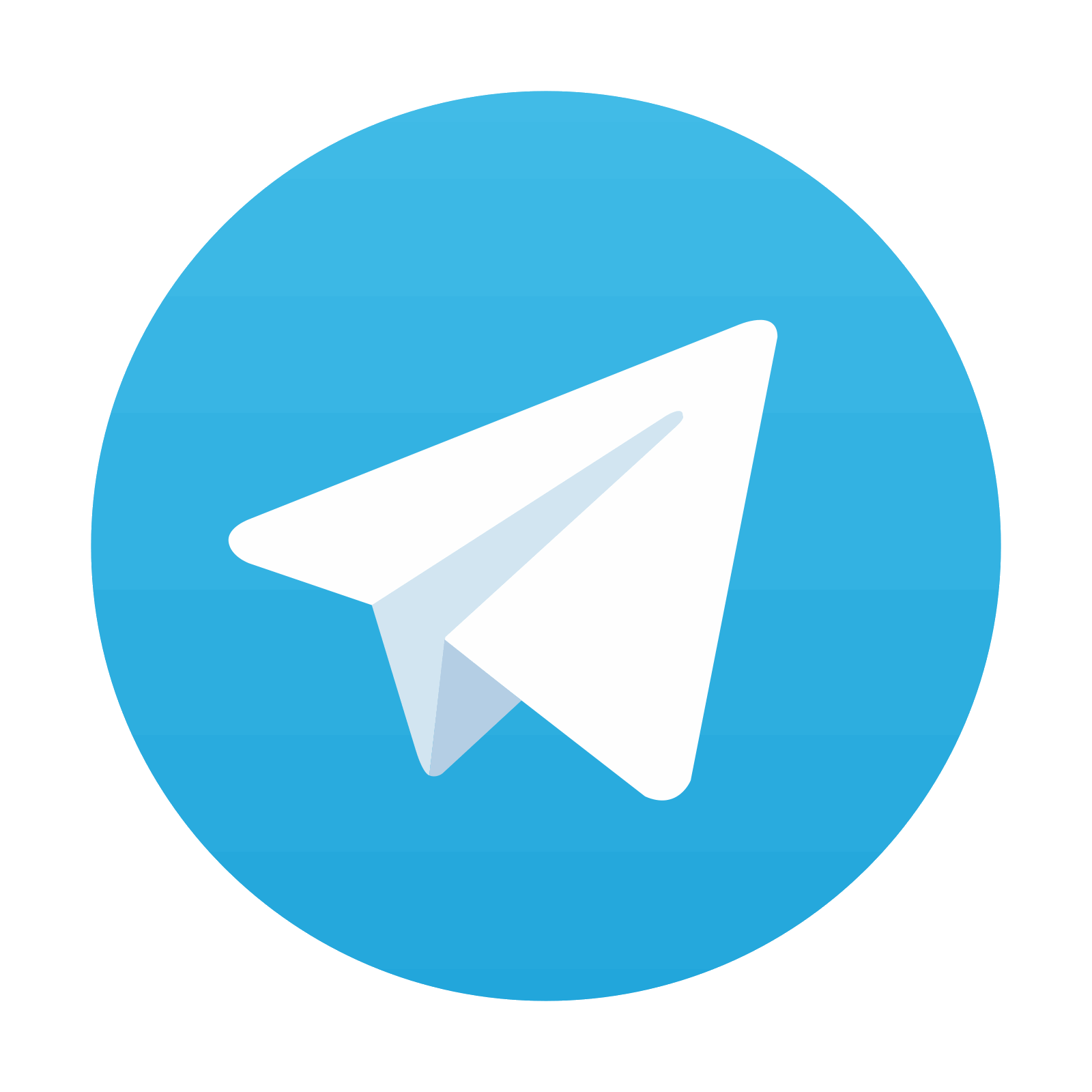
Stay updated, free articles. Join our Telegram channel
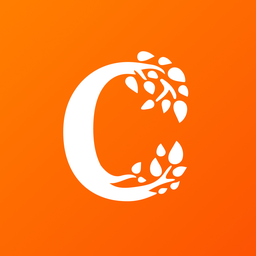
Full access? Get Clinical Tree
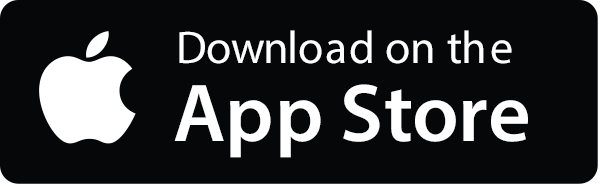
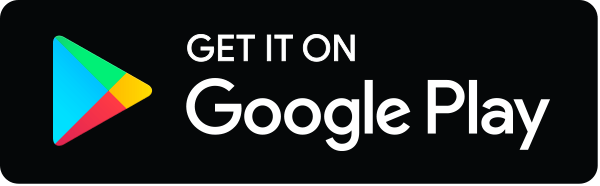