In the surgical management of solid tumors, adequacy of tumor resection has implications for local recurrence and survival. The standard method of intraoperative identification of tumor margin is frozen section pathologic analysis, which is time-consuming with potential for sampling error. Intraoperative tumor visualization has the potential to significantly improve surgical cancer care across disciplines, by guiding accuracy of biopsies, increasing adequacy of resections, directing adjuvant therapy, and even providing diagnostic information. We provide an outline of various methods of intraoperative tumor visualization developed to aid in the real-time assessment of tumor extent and adequacy of resection.
Key points
- •
Intraoperative tumor visualization has the potential to significantly improve surgical cancer care by improving adequacy of resection, decreasing resection of normal tissue and structures, and directing adjuvant therapy.
- •
Various techniques for intraoperative tumor imaging exist, including optical fluorescent imaging, high-frequency ultrasound, optical coherence tomography, optoacoustic imaging, confocal microscopy, elastic scattering spectroscopy, Raman spectroscopy, and radiofrequency spectroscopy; however, no single technique has yet been perfected.
- •
Optical fluorescence imaging has been further refined with the use of near-infrared techniques and selective probes, improving the sensitivity and accuracy of intraoperative images.
Introduction
The surgical oncologist is tasked with a great responsibility, with little margin for error. One must localize the tumor and resect it, preferably en bloc, with as little morbidity and contamination to surrounding tissues as possible. Adequacy of resection has implications on local recurrence and survival for many tumor types. Incorrect estimation of tumor resection margins may result in incomplete excision of disease, or increased morbidity with resection of excess normal tissue. Various methods of intraoperative tumor visualization have been, and are being, developed to aid in the real-time assessment of tumor extent and adequacy of resection. Better understanding of the resection margins has implications for adjuvant treatment as well. Knowledge of an increased or decreased risk of local recurrence may inform decisions about adjuvant therapies, such as radiation.
Assessment of the margins of resected tumor is a surrogate measure for the ultimate question: whether or not tumor remains in the patient. Analysis is performed via gross and histologic evaluation of the resected tissue, and is a lengthy and imperfect process. The standard and most widely used method of intraoperative identification of tumor margin is frozen section pathologic analysis. Although it is an accurate way of identifying tumor in the sampled tissue, only a small percentage of the margin of resected tissue is actually analyzed. Thus, there is great potential for sampling error both from random sampling of the resected tissue, as well as heterogeneity of the tumor itself. Furthermore, the process is time-consuming and correlation of orientation/localization between the tumor and tumor bed are distorted after excision. Final histologic analysis of complete tumor margins can take up to 5 to 7 days. If margins are found to be positive, the patient may be subjected to reoperation, adjuvant treatments, or both.
Multiple methods for intraoperative tumor visualization have been developed and continue to be refined. Many of these advances have been driven by brain and head and neck cancers due to the morbidity associated with tissue resection, as well as breast cancers with the increase in breast-conserving surgical techniques. However, a mature technique for intraoperative tumor visualization would benefit surgeons and their patients across all disciplines, guiding accuracy of biopsies, increasing adequacy of resections, and in some instances even providing diagnostic information.
Introduction
The surgical oncologist is tasked with a great responsibility, with little margin for error. One must localize the tumor and resect it, preferably en bloc, with as little morbidity and contamination to surrounding tissues as possible. Adequacy of resection has implications on local recurrence and survival for many tumor types. Incorrect estimation of tumor resection margins may result in incomplete excision of disease, or increased morbidity with resection of excess normal tissue. Various methods of intraoperative tumor visualization have been, and are being, developed to aid in the real-time assessment of tumor extent and adequacy of resection. Better understanding of the resection margins has implications for adjuvant treatment as well. Knowledge of an increased or decreased risk of local recurrence may inform decisions about adjuvant therapies, such as radiation.
Assessment of the margins of resected tumor is a surrogate measure for the ultimate question: whether or not tumor remains in the patient. Analysis is performed via gross and histologic evaluation of the resected tissue, and is a lengthy and imperfect process. The standard and most widely used method of intraoperative identification of tumor margin is frozen section pathologic analysis. Although it is an accurate way of identifying tumor in the sampled tissue, only a small percentage of the margin of resected tissue is actually analyzed. Thus, there is great potential for sampling error both from random sampling of the resected tissue, as well as heterogeneity of the tumor itself. Furthermore, the process is time-consuming and correlation of orientation/localization between the tumor and tumor bed are distorted after excision. Final histologic analysis of complete tumor margins can take up to 5 to 7 days. If margins are found to be positive, the patient may be subjected to reoperation, adjuvant treatments, or both.
Multiple methods for intraoperative tumor visualization have been developed and continue to be refined. Many of these advances have been driven by brain and head and neck cancers due to the morbidity associated with tissue resection, as well as breast cancers with the increase in breast-conserving surgical techniques. However, a mature technique for intraoperative tumor visualization would benefit surgeons and their patients across all disciplines, guiding accuracy of biopsies, increasing adequacy of resections, and in some instances even providing diagnostic information.
Traditional imaging techniques
Traditional imaging techniques, such as MRI and ultrasound. have been studied in brain tumor resection and breast-conserving surgery, respectively. Studies have shown increased rates of complete tumor resection without increased neurologic deficits, and improved survival rates with intraoperative MRI-guided resection of gliomas. Although safe and effective, there are disadvantages to this technique. For one, it requires the surgeon to interpret the imaging and correlate the location of the lesion from the image to the tumor bed. Second, previously treated areas may have treatment effects or postoperative changes that affect the MRI image and decrease the accuracy of this technique. Third, obtaining an MRI can be time-consuming, resulting in significant surgical disruptions and prolonged operative time. Finally, there is considerable investment required to create operative suites with the ability to accommodate the logistical needs of the MRI magnet. Ultrasound has fewer logistical pitfalls than MRI, and has been used to localize the tumor, guide resection, and confirm completeness of excision. Studies show ultrasound-guided resection of breast lesions to have improved resection margins and decreased excision volumes than traditional techniques, such as palpation guided, wire localization, or quantitative radionucleotide-guided localization. However, the resolution of ultrasound is poor, and similar to other structural imaging modalities, the accuracy is affected by previously treated surgical fields.
Computer-assisted navigation using fiducial markers that are calibrated to the patient’s anatomy and cross-sectional (computed tomography [CT] or MRI) imaging can be used for indirect tumor imaging. Calibrated pointers, or surgical instruments (ie, osteotome, burr, cautery) can localize the instrument within the patient’s cross-sectional images on a monitor in real time. This can be used to correlate to the planned resection margin, to the tumor, or to other vital anatomic structures. Computer-assisted navigation systems have gained popularity in pelvic resections for technically demanding, complex multiplanar osteotomies, and joint-preserving excisions. They have been shown to improve excision accuracy with adequate resection margins ( Fig. 1 ). However, accuracy with navigation of soft tissue tumors is poor because of inability to place a stable fiducial, and disruption of the localizers may result in misguided navigation.
Optical fluorescent imaging
Optical imaging is based on fluorescence variation between normal and tumor tissue, which may be driven by intrinsic tissue properties or exogenously infiltrated optical agents. It requires 3 basic components: a fluorophore agent that will emit light when excited (may be endogenous to tissues or administered exogenously), a light source (at a wavelength that will excite the fluorophore), and a camera system (that will capture the fluorescence emitted from the excited fluorophore). There are many different variations of optical imaging techniques that will be discussed in more detail, but the basic method is described as follows :
- 1.
A light source emits light at a specific wavelength known to excite the chosen fluorophore.
- 2.
The fluorophore may be endogenous to tissues (autofluorescence) or administered to the patient (fluorescent probe). Exogenous probes can be administered preoperatively via intravenous (IV) or local infiltration, or applied topically onto the tumor bed or resected tissue intraoperatively.
- 3.
This light wavelength (photon) travels into the tissue and is absorbed by the fluorophore. It is subjected to reflection, refraction, and scattering as it penetrates the tissues.
- 4.
The fluorophore enters a state of excitation for a fraction of a second (depending on the lifetime of the fluorophore).
- 5.
When the fluorophore returns to its ground state, it emits the photon at another given wavelength of light.
- 6.
This light wavelength travels out of the tissue (again subjected to reflection, refraction, and scattering) and is captured and recorded by the camera system.
Autofluorescence
This technique uses a light source in the UV to visible range, and exploits differences in the inherent optical properties between normal and pathologic tissue, resulting in differences in light scatter and autofluorescence. One such technique, described by Wilke and colleagues, is based on the premise that tumor tissue has a different profile of β-carotene and hemoglobin from surrounding normal tissue. Evaluation of the margins of resected tissue correctly identify 79% of histologically positive margins by this technique. Autofluorescence also has been used to assess the margins of surgical beds during resection of oral cavity cancers. In this method, the destruction of collagen matrix and increased vascularity within the tumors resulted in a decrease in autofluorescence recorded by a handheld camera device.
Advantages
- •
Real-time, intraoperative visualization of tumor
- •
Takes advantage of tissue property differences without administration of exogenous fluorophores
- •
Can image tumor or tumor bed
- •
Wide field imaging
- •
Can be used to both localize tumor and assess tumor bed for residual disease, or assess excised tissue for status of margins
Disadvantages
- •
Relatively low tumor-to-background signal ratio
- •
Blood pooling and cauterized tissue in the surgical field increases the scattering/absorption of light, and may falsely decrease the fluorescent signal
- •
Tissue optical properties may be altered by neoadjuvant cancer therapies
- •
Changes in tissue morphology after resection may alter the evaluation of margins in excised tissue
- •
Shallow depth of penetration (∼2 mm)
Near-Infrared Fluorescent Imaging
The light source chosen for optical imaging techniques may be in the visible (400–800 nm) or the near-infrared (NIR; 700–900 nm) spectrum, based on the excitation properties of the given fluorophore. Compared with visible light, NIR light has many advantages. First, it has better penetration of soft tissues (on the order of centimeters compared with the millimeters of penetration by visible light). Second, NIR fluorescence techniques can use an exogenous fluorescent probe that localizes to tumor cells to increase the fluorescent signal produced by tumor tissue. NIR light generates minimal autofluorescence by the surrounding tissues in the background, resulting in increased tumor “signal”-to-background “noise” ratio with use of exogenous fluorescent probes. This increases the sensitivity of NIR compared with visual spectrum fluorescence in tumor detection. NIR systems can be free-standing, adapted into intraoperative microscopes, laparoscopes, and robots, or small handheld probes ( Fig. 2 ). Fluorescence molecular tomography uses complex mathematical formulas to derive 3-dimensional images from optical fluorescent techniques. These fluorescent renditions can be superimposed onto CT or MRI images to create an anatomic reference with high sensitivity and resolution.
Advantages
- •
Greater depth of field
- •
Decreased scattering/reflectance, and use exogenous fluorescent probes increase the fluorescent “signal”
- •
Decreased autofluorescence reduces the background “noise”
- •
Decreased absorption by hemoglobin and lipid improves optimal use in surgical field
Disadvantages
- •
Not visible with the naked eye: requires a camera system able to capture light and translate onto an imaging screen; however, newer hand-held systems have greatly improved the convenience of use
Nontargeted Fluorescent Probes
Optical imaging can be performed with fluorescent probes that are administered to the patient and preferentially infiltrate into the tumor tissue. Nontargeted probes are based on the enhanced permeability and retention (EPR) effect. EPR exploits properties of the tumor, such as increased vascularity, leaky capillary membranes, and abnormal lymphatic drainage, which result in increased delivery and retention of the exogenous fluorophores to the target tissue. These non–tumor specific agents include fluorescein, indocyanine green dye (ICG), cresyl violet, toluidine blue, Lugol iodine, and acridine orange. These can be administered intravenously, applied topically, or injected in or near the tumor. Local injection, termed diffusion molecular retention, typically uses pegylated probes to further enhance interstitial diffusion and tumor uptake. This results in slowed uptake and clearance by vascular channels, prolonging interstitial diffusion and further decreasing systemic circulation.
Advantages
- •
ICG, the predominant fluorophore used in NIR fluorescence, is rapidly cleared by the liver and has low rates of toxicity
- •
Camera devices used in NIR techniques are designed to capture ICG fluorescence, but also are able to image other fluorophores with similar excitation and emission profiles as newer tumor specific agents become approved for clinical use
Disadvantages
- •
Contrast allergy is a relative contraindication to ICG use
- •
Time sensitive due to the rapid clearance of ICG
- •
Relatively low signal-to-background ratio
Targeted Fluorescent Probes
Recent advancements have led to the development of probes linking a fluorescent agent to a tumor-specific target, many of which are based on existing cancer-targeted therapies. These targets, as outlined in Table 1 , are based on the premise that gene dysregulation of tumor cells leads to increased angiogenesis, metabolism, and expression of growth signal receptors that can be exploited as biomarker targets for tumor-specific probes.
Unique Tumor Property | Target | Example |
---|---|---|
Unlimited replication potential | Increased metabolism | IRDye800CW 2-deoxyglucose (Li-Cor Bioscience, Lincoln, NE, USA) |
Increased angiogenesis | Increased hemoglobin concentration | Spectroscopy |
VEGF receptor | NIRF-labeled bevacizumab (Genentech, Inc., San Francisco, CA, USA) | |
Ανβ3-Integrin | IRDye800CW RGD, IntegriSense (PerkinElmer, Waltham, MA, USA) | |
Increased vascular permeability | IRDye800CW PEG, AngioSense (PerkinElmer) | |
Tissue invasion and metastasis | Matrix metalloproteinases | MMPSense (PerkinElmer, Waltham, MA, USA) |
Cathepsins | ProSense (PerkinElmer) | |
Upregulation of growth signals | EGF receptor | IRDye800CW EGF, NIRF-labeled cetuximab (ImClone LLC, Branchburg, NJ, USA), NIRF-labeled trastuzumab (Genentech, Inc.) |
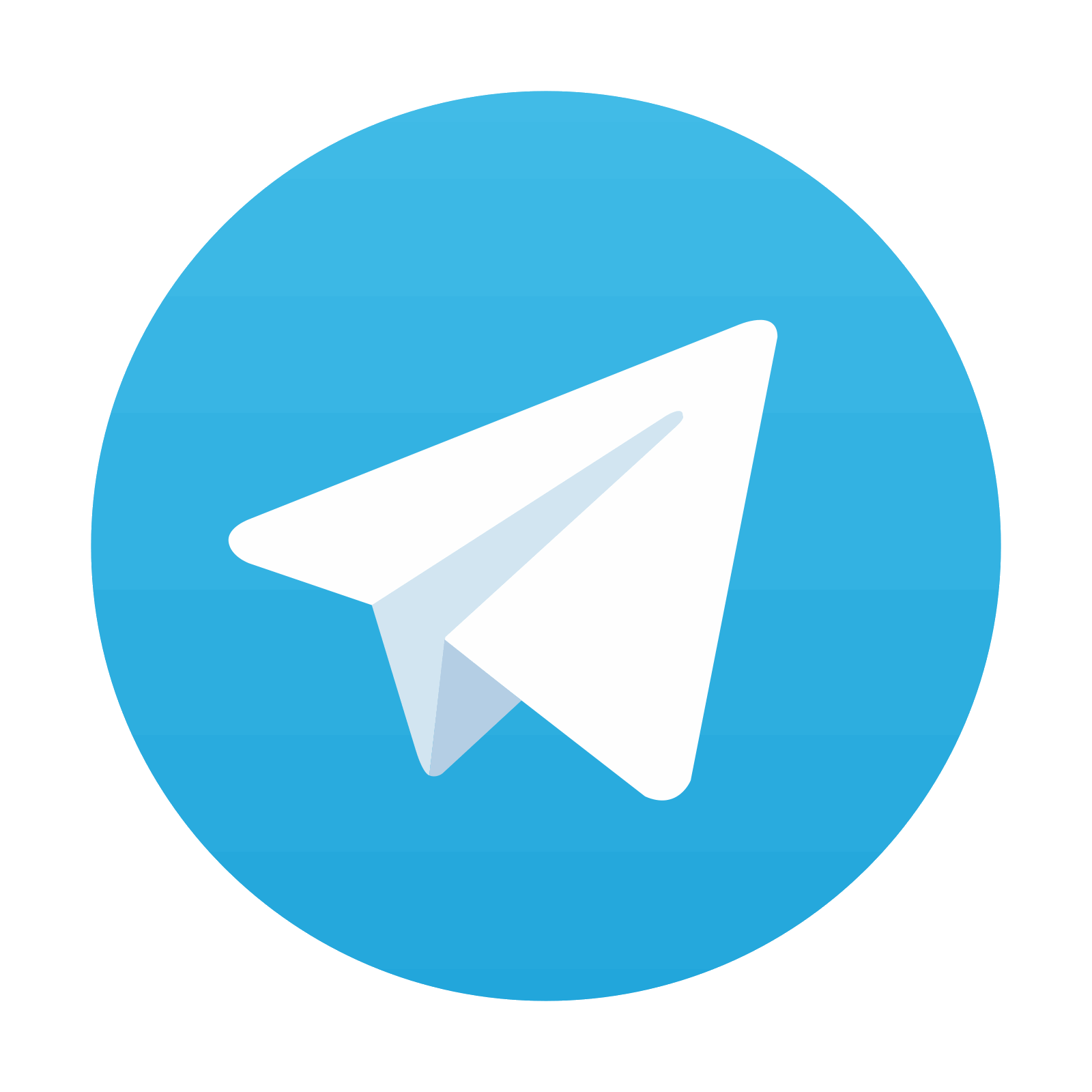
Stay updated, free articles. Join our Telegram channel
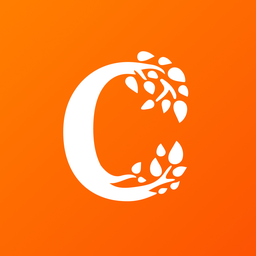
Full access? Get Clinical Tree
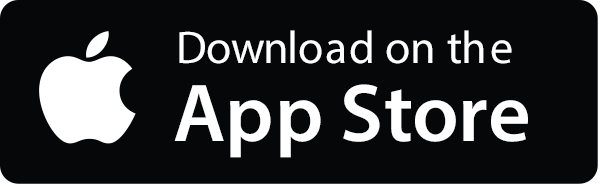
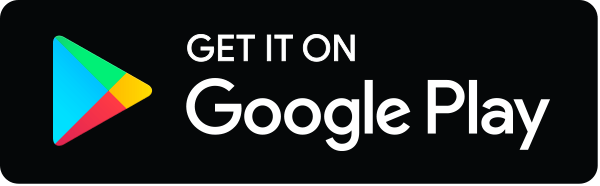
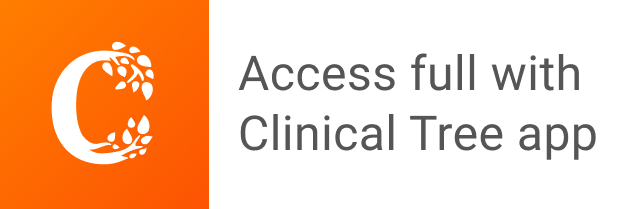