making functional molecules called proteins. Study of these genes (including other components such as enhancers and small ribonucleic acids or RNAs) is called genetics, which also encompasses other components such as heredity and genetic variation. Proteins alone or in complexes perform a variety of cellular functions. With the advancement of technology and discovery of new phenomena, the concept of a gene continues to be refined. For instance, regulatory regions of a gene may be far away from the coding regions, and coding regions may consist of several split exons (a segment of a DNA molecule containing information coding for peptides). The domain of genetics is broad and encompasses several conceptual frameworks that include but are not limited to genomics, transcriptomics, proteomics, heredity, evolutionary genetics, and genetic diseases (Figure 2).
![]() FIGURE 1 Illustration showing nucleotide making up DNA, which is stowed in chromosomes within the nucleus of cells that reside in every tissue of the body. |
messenger RNA molecules in a cell or population of cells and contains the full information about all RNA transcribed by the genome in a specific tissue or cell type, at a certain developmental stage, and under explicit pathophysiological circumstances. For instance, we know that osteocytes are derived from osteoblast lineage cells that become progressively embedded in mineralized bone matrix in the adult skeleton.5 These cells are morphologically, functionally, and genetically unique, due in part to the underlying expression of selective gene subsets that characterize the osteocyte phenotype. Although substantial changes in gene expression take place during osteoblast to osteocyte transition, the majority of the transcriptome remains qualitatively osteoblast-like.
two types: synonymous and nonsynonymous. Synonymous SNPs do not affect the protein sequence, whereas nonsynonymous SNPs change the amino acid sequence of a protein. SNPs that are not in protein-coding regions may still affect gene splicing, transcription factor binding, messenger RNA degradation, or the sequence of noncoding RNA. Gene expression affected by this type of SNP is referred to as an eSNP (expression SNP). Besides SNPs, there also exist several other forms of genetic variations such as copy number variations (CNVs). A CNV is a type of structural change (deletions, insertions, duplications, and complex multisite variants) in the genome in which the sections of genome (a DNA segment of 1 kb or large) are repeated in the form of gain or loss of segments of genomic DNA relative to a reference sequence.11 CNVs are as important a component of genomic diversity as SNPs. Although SNPs are variations at the level of single nucleotide and account for less than 1% of human genome, CNVs affect regions >1 kb and cover roughly 35% of genome.12
reverse disease progression. Genomic studies have provided important insights into the biologic mechanisms underlying differences in susceptibility or resistance of an individual to musculoskeletal diseases such as RA, OA, and systemic lupus erythematosus. It has been estimated that 39% to 78% cases of OA can be attributed to genetics.19 Genetics also plays a crucial role in drug development. For example, pharmacogenomics involves genetic variants that impact expression of genes affecting drug pharmacodynamics such as absorption, distribution, action, metabolism, and toxicity of a drug. The term pharmacogenomics is often used interchangeably with the term pharmacogenetics. Pharmacogenetics refers to inherited genetic differences in drug metabolic pathways. It also pertains to other pharmacological principles such as enzymes, messengers, and receptors, which can affect individual responses to drugs, in terms of both therapeutic benefits and adverse effects.
TABLE 1 Significant SNPs Associated With Hip, Knee, and Hand OA | ||||||||||||||||||||||||||||||||||||||||||||||||||||||||||||||||||||||||||||||||||||||||||||||||||||||||||||||||||||||||||||||||||||||||||||||||||||||||||||||||||||||||||||||||||||||||||||||||||||||||||||||||||||||||||||||||||||||||||||||||||||||||||||
---|---|---|---|---|---|---|---|---|---|---|---|---|---|---|---|---|---|---|---|---|---|---|---|---|---|---|---|---|---|---|---|---|---|---|---|---|---|---|---|---|---|---|---|---|---|---|---|---|---|---|---|---|---|---|---|---|---|---|---|---|---|---|---|---|---|---|---|---|---|---|---|---|---|---|---|---|---|---|---|---|---|---|---|---|---|---|---|---|---|---|---|---|---|---|---|---|---|---|---|---|---|---|---|---|---|---|---|---|---|---|---|---|---|---|---|---|---|---|---|---|---|---|---|---|---|---|---|---|---|---|---|---|---|---|---|---|---|---|---|---|---|---|---|---|---|---|---|---|---|---|---|---|---|---|---|---|---|---|---|---|---|---|---|---|---|---|---|---|---|---|---|---|---|---|---|---|---|---|---|---|---|---|---|---|---|---|---|---|---|---|---|---|---|---|---|---|---|---|---|---|---|---|---|---|---|---|---|---|---|---|---|---|---|---|---|---|---|---|---|---|---|---|---|---|---|---|---|---|---|---|---|---|---|---|---|---|---|---|---|---|---|---|---|---|---|---|---|---|---|---|---|---|
|
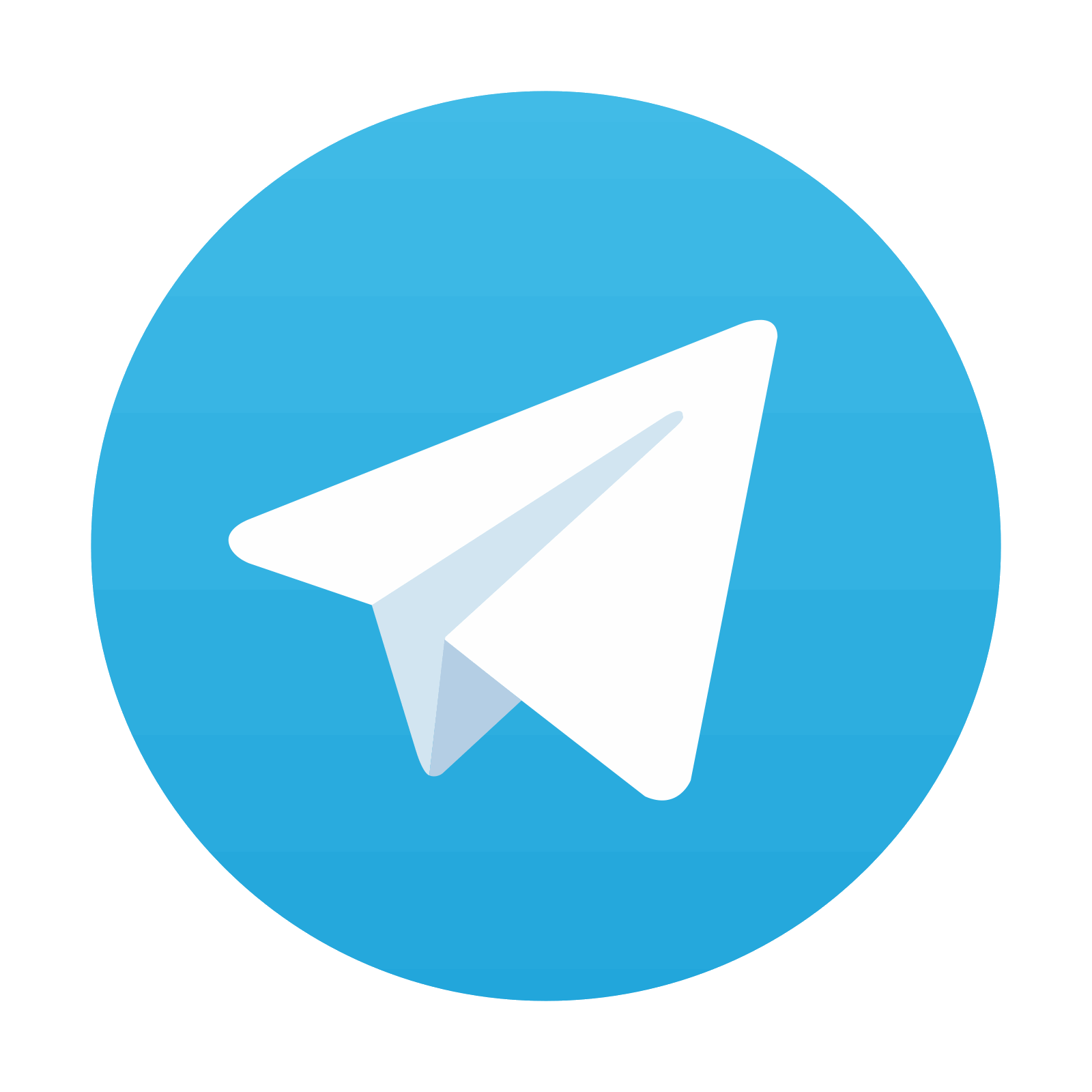
Stay updated, free articles. Join our Telegram channel
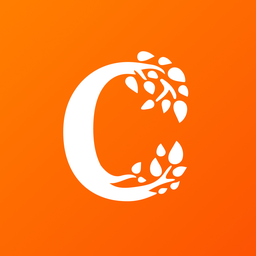
Full access? Get Clinical Tree
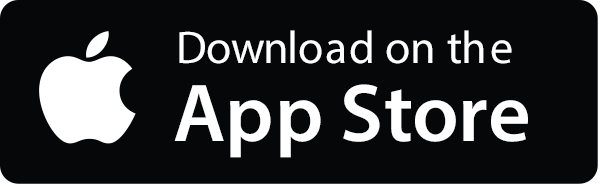
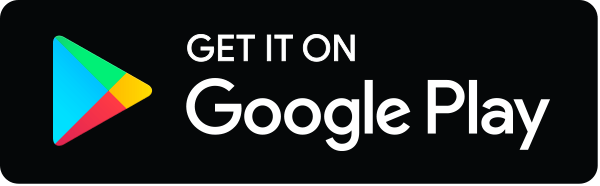