26
Gene Therapy in the Treatment of Cartilage Injury
Limitations of Current Treatments of Cartilage Injury
The specialized architecture and limited repair capacity of articular cartilage, coupled with the high physical demands placed upon this tissue, make it exceedingly difficult to treat cartilage injury medically. As outlined in other chapters of this book, several surgical treatment options are available for resurfacing chondral and osteochondral lesions. “Traditional” resurfacing procedures such as debridement, abrasion arthroplasty, drilling, and microfracture aim to promote natural fibrocartilage repair.1 As fibrocartilaginous scar tissue has inferior mechanical and physical properties compared with normal articular cartilage, modern “resurfacement” tries to achieve a more hyaline-like cartilage repair. Such procedures include transplantation of periosteum or perichondrium, autologous osteochondral transfers, autologous chondrocyte implantation, and tissue engineering approaches, which deliver a space-filling entity composed of cells with or without matrix. Despite these advances, most surgical interventions result in improvement of clinical symptoms such as pain relief, but none of the current treatment options have regenerated long-lasting hyaline cartilage tissue to replace damaged cartilage.1
Cartilage Injury Versus Disease
Many who study cartilage repair fail to draw distinctions between lesions resulting from acute injury and those resulting from disease. Acute cartilage injury often arises in an otherwise healthy joint; the patient may be young, and the focal defect will likely require a localized treatment. In contrast, damage to cartilage that arises from an underlying disease process, such as in osteoarthritis (OA), where the patient is likely to be older, may require the treatment of the entire articulating region. In this case, repair of the lesion may provide symptomatic relief and delay of the progression of symptoms, but unless the underlying disease is also treated effectively, any improvement is likely to be temporary. Gene transfer approaches might be adapted for the treatment of both localized cartilage injury and diseased cartilage, but these should be recognized as different entities.
Why Can Gene Therapy Be Important for Cartilage Repair?
With the completion of the Human Genome Project, a new era of gene-based therapies is evolving, including applications in the field of orthopaedics. Beyond the goal of replacement of defective genes, gene therapy has become a tool for delivering individual proteins and other gene products to specific tissues and cells. It is conceivable that the above-stated limitations of cartilage repair may be overcome by adapting gene transfer technologies. In particular, it should be possible to develop techniques for transferring genes encoding the necessary gene products to the appropriate sites in the joint, and for expressing those genes locally for the intervals of time needed to achieve cartilage repair. Data are beginning to emerge that suggest delivery and expression of certain genes can influence a repair response toward the synthesis of normal articular cartilage in vivo. This chapter gives a systematic overview of the current status of gene delivery for cartilage healing, and presents some of the remaining challenges in the development of successful gene-based cartilage repair techniques.
Which Proteins Aid Cartilage Repair?
Research advances in cellular, molecular, and developmental biology have led to the identification of a variety factors that might be useful in the treatment of cartilage disease and injury. These molecules have a broad range of activities, among which are the capacity to induce the synthesis and deposition of cartilage extracellular matrix components by chondrocytes, stabilization of the extracellular matrix, induction of chondrogenesis by mesenchymal progenitor cells, and the ability to inhibit inflammatory processes (Table 26–1). It is thought that the coordinated administration of the appropriate protein cocktail to chondrocytes, chondroprogenitors, or synovial lining cells could be used to improve treatment of injured or diseased cartilage. This could occur either by fostering differentiation into and/or maintenance of the appropriate chondrocyte phenotype for synthesis of repair tissue that functionally resembles normal articular cartilage, or by protecting the cartilage from inflammatory and degradative stimuli.2
Potentially useful in this respect are members of the transforming growth factor-β (TGF-β) superfamily, including TGF-β1, -β2, and -β3, several of the bone morphogenetic proteins (BMPs), insulin-like growth factor-I (IGF-I), fibroblast growth factors (FGFs), and epidermal growth factor (EGF), among others (reviewed in Hickey et al3). Other secreted proteins, such as Indian (IHH) or sonic (SHH) hedgehog play key roles in regulating chondrogenic hypertrophy4 and may also prove beneficial for modulating chondrogenesis of grafted cells. Another class of biologics that may be useful in cartilage repair is the transcription factors that promote chondrogenesis or the maintenance of the chondrocyte phenotype. Sox9 and related transcription factors such as L-Sox5, and Sox6 have been identified as essential for chondrocyte differentiation and cartilage formation.5 Signal transduction molecules, such as SMADs, are also known to be important regulators of chondrogenesis.6
Gene Product | Examples | |
---|---|---|
Stimulator of matrix synthesis | IGF-I, BMPs, TGF-βs | |
Stimulator of chondrogenic differentiation | Cartilage growth and differentiation factors: BMPs, TGF-βs, IGF-I, FGFs, EGF, SHH, IHH | |
Transcription factors: L-SOX5, SOX6, SOX9 | ||
Signal transduction molecules | SMADs | |
ECM components | Type II procollagen | |
Cytokines and cytokine antagonists | Proinflammatory cytokine inhibitors IL-1Ra, sIL-1R, sTNFR | |
Antiinflammatory cytokines IL-4, IL-10, IL-13 | ||
Apoptosis inhibitors | Bcl-2 |
As an alternative to stimulating expression of endogenous chondrogenic genes, delivery and expression of complementary DNAs (cDNAs) encoding specific extracellular matrix (ECM) components may be used for maintenance of the proper hyaline cartilage phenotype.7 To treat cartilage loss due to diseases such as OA or rheumatoid arthritis (RA), inhibition of the actions of certain proinflammatory cytokines, such as interleukin-1 (IL-1) and tumor necrosis factor-α (TNF-α) may be required. These proteins, synthesized by synovial cells and chondrocytes, are found at elevated levels in arthritic joints and are important mediators of cartilage degradation. Administration of mediators that are antiinflammatory or immunomodulatory, such as IL-1 receptor antagonist (IL-1Ra), soluble receptors for TNF (sTNFR) or for IL-1 (sIL-1R), IL-4, or IL-10 may be effective in reducing cartilage loss.8 Moreover, apoptosis inhibitors such as bcl-2 and nitric oxide (NO) antagonists could be employed to maintain chondrocyte populations.
Furthermore, various combinations of candidate growth factors may also be envisaged. For example a dual-axis therapy using an anabolic growth factor like TGF-β or IGF-I, and an inhibitor of the catabolic action of inflammatory cytokines such as IL-1Ra, has potential synergistic effects in the repair process.
However, as already mentioned, the clinical application of recombinant proteins is hindered by several obstacles. In vivo, many proteins have short half-lives and thus are difficult to maintain at functional concentrations for periods sufficient to facilitate an effective repair, making repeated administration or methods for timed-release necessary. Certain proteins, such as transcription factors or signal transduction molecules, function completely intracellularly, and because cells cannot normally import these molecules, they cannot be readily delivered in soluble form.
Gene Transfer as a Protein Delivery System
Gene transfer offers an alternative approach to protein delivery that may satisfactorily overcome the limitations of conventional methods.2 By delivering cDNAs that code for various chondrogenic or therapeutic proteins to specific target cells and providing for sustained synthesis of the transgene products, the genetically modified cell is converted to a small factory for protein production (Fig. 26–1). Through the localized delivery of gene transfer vectors or genetically modified cells to specific sites of damage, protein synthesis can be concentrated at the site of injury or disease with minimal collateral exposure of nontarget tissues.
FIGURE 26–1 Gene transfer as protein delivery system. A therapeutic gene is inserted into a vector, which can be viral or nonviral in nature. The vector delivers its genetic material to the cell, where it is translocated to the nucleus of the host cell. In the nucleus the DNA stays either episomal (nonintegrating vector) or inserts its genetic material into chromosomes of the host cells (integrating vector). The complementary DNA (cDNA) encoding the protein then serves as a template for synthesis of the corresponding messenger ribonucleic acid (mRNA) by the endogenous transcriptional machinery of the cell. The mRNA leaves the nucleus and is translated into protein by the ribosomal machinery and, if a secreted protein, is released from the cell. In this way, the genetically modified host cell is converted into a small factory for protein production. Protein synthesis can be localized to the site of injury or disease, with minimal collateral exposure of nontarget tissues.
There are various ways to deliver exogenous cDNAs to joint tissues for treatment of cartilage lesions. In devising a useful strategy, several factors must be taken into account, including the specific disease, the extent of cartilage pathology, and the biologic activity of the gene product. A key component to any gene therapy application is a vector that efficiently delivers the cDNA of interest to the target cell, and enables transgene expression of a suitable level and duration to affect the desired biologic response. Furthermore, an understanding of the natural behavior of the target cell, such as its half-life, rate of division, and accessibility to the vector, is also essential to the effectiveness of the procedure.
Nucleic Acids that Directly Regulate Gene Expression
In addition to genes that encode messenger RNA (mRNA) leading to the synthesis of specific proteins, there are also those that give rise to RNA but no protein. Of particular interest are species of nucleic acids with the ability to directly regulate activities of other genes, and which may be harnessed for disease therapy and tissue regeneration by gene transfer methods.9 These include antisense and “decoy” oligonucleotides, ribozymes, and small interfering RNAs (siRNAs). Antisense oligodeoxynucleotides (ODN) are short single-stranded nucleic acids that hybridize to specific mRNA molecules, resulting in the formation of RNA/DNA duplexes that are cleaved by nucleases leading to subsequent block of gene expression.10 Decoy oligonucleotides are double-stranded nucleotides that are recognized and bound specifically by critical molecules, such as transcription factors. Thus, these decoys can completely inhibit the activities of proteins, necessary for the expression of particular endogenous genes.11 Ribozymes are catalytic RNA molecules that cleave RNA substrates in a sequence-specific fashion. Various entities exist, and initial gene therapy clinical trials are currently underway, using this technique to treat various infectious diseases.9 RNA interference (RNAi) is the term used to describe the blocking of gene expression by the introduction of small fragments of double-stranded RNA (dsRNA) into the cell cytoplasm. The mechanism involves the conversion of the added dsRNA into short interfering RNAs (siRNAs) that direct ribonucleases to homologous RNA targets.12 Its actions are highly specific and very efficient in mammalian cells.
These techniques have potential use in diseases where overexpression of a normal or mutated gene contributes to pathology. For example, it might be beneficial for any cartilage regeneration process to limit expression of osteogenic genes such as osteocalcin or Cbfa1, or genes such as noggin that antagonize the actions of certain BMPs. The major advantage of the delivery of such genes is that they directly regulate gene expression, and are not translated into proteins, thus avoiding the potential risk of dissemination of stimulatory factors to nontarget sites and immune responses to allo- or xenogenic proteins.
Gene-based methods currently being explored for the treatment of articular cartilage injury range from those as simple as direct delivery of a vector to a defect, to synthesis of cartilaginous implants through genetically augmented tissue engineering procedures. In the next few paragraphs we give an overview of the properties of commonly used vectors in gene therapy applications and discuss their use in the context of the different delivery strategies to joint tissues.
Choosing a Strategy for Gene Delivery
Because exogenous cDNAs are not spontaneously taken up and expressed by cells, they have to be transferred to cells with the aid of a vector. The most commonly used and most effective methods to insert DNA into target cells are by way of a viral vector, which is termed “transduction.” An alternative to the viral gene transfer systems are the nonviral methods of delivering DNA, termed “transfection,” which are typically less efficient.
Viral Vectors for Orthopaedic Applications
To convert a virus into a gene transfer vector, the parts of the viral genome that enable it to replicate and cause disease are removed and replaced with therapeutic genes. This results in recombinant viruses that retain their infectivity and ability to transfer genes, minus any virulence they might have possessed. Vectors derived from several types of viruses have been used to transfer genes to joints, and have been reviewed extensively.13 Some of the most important characteristics of each these viral vectors are summarized in Table 26–2. Viral vectors are generally categorized according to whether their genomes integrate into the host cells chromosomes (retroviruses, lentiviruses) or whether they do not integrate but persist in the cell nucleus predominantly as episomal DNA [recombinant adeno-associated virus (AAV), adenovirus, and herpes virus vectors]. Episomal DNA can be lost with time, especially if the cell divides. This distinction is one important determinant for the suitability of each vector system for a specific application; nonintegrating vectors can be harnessed for applications where transient expression of the transgene is desired, but integrating vectors are, at present, the tools of choice if stable genetic alteration needs to be maintained in dividing cells.
Adenoviral Vectors
Wild-type serotype 5 adenovirus causes mild respiratory and eye infections in humans. Vectors based on this virus are nonintegrating and have the advantage of being able to infect dividing and nondividing cells with excellent efficiency. Recombinant adenoviral vectors are easy to construct and purify14 and can be grown in high titer to greater than 1014 particles per milliliter. Adenovirus vectors can be divided into three general categories—first generation, second generation, and gutless or high capacity—depending on the number of viral genes which have been inactivated. First-generation adenoviral vectors, deficient for one or two viral early genes (E1 and E3), have been used in several clinical trials (reviewed in Thomas et al15). First-generation adenovirus vectors transduce chondrocytes, synoviocytes, and chondroprogenitor cells very efficiently.
There are several disadvantages, however, associated with first-generation adenoviral vectors. Despite deletion of some of the critical early-phase genes, low levels of the remaining viral proteins are expressed. This results in an immune response to the virally infected cell in vivo, which may ultimately lead to clearance of the infected cells. Further deletion of genes E2 and E4 in second-generation vectors reduces but does not eliminate the expression of viral genes. “Gutted” adenoviral vectors, also termed high-capacity adenoviral vectors, in contrast, contain no viral coding sequences and thus avoid this problem, while also enabling packing of up to 35 kilobase (kb) of exogenous DNA. Unfortunately, these vectors are considerably more difficult to manufacture and purify.13
Adeno-Associated Viral Vectors
Adeno-associated viruses (AAVs) are single-stranded, nonenveloped, small DNA viruses that require co-infection with a helper virus such as an adenovirus or a herpes simplex virus for replication. Wild-type AAV is not known to cause any disease in humans, and inserts its genetic material into a specific site on chromosome 19 of the host cell. The virus infects a wide variety of dividing and nondividing cells, but with varying levels of efficiency. Recently, recombinant AAV vectors have been designed that allow for generation of large scale, high-titer, and helper-virus free preparations. Further, the design of high-efficient self-complementary AAV-based vectors avoids problems associated with second-strand synthesis.16 These AAV vectors are mainly nonintegrating and persist in many cell types as large episomal concatamers. Recombinant AAV vectors encode no viral gene products and may not generate neutralizing immune reactions. However, the main disadvantages are the small “cargo size” of ~4 kb, difficult production, and variable transduction efficiency. The use of AAV vectors to transfer genes to joints has been addressed with mixed results so far.17 Synovium appears only modestly susceptible to transduction with recombinant AAV vectors, but chondrocytes are more readily transduced. There is evidence that the small size of AAV might allow it to transduce chondrocytes in situ, unlike the case with other viral vectors.
Herpes Simplex Virus Vectors
The herpes simplex virus (HSV) is a large enveloped, double-stranded DNA virus that can be engineered to hold up to 40 kb of foreign DNA, and stocks of 109 to 1012 infectious particles per milliliter can be generated. HSV can be used to infect both dividing and nondividing cells of many types. Major disadvantages are the difficulty in creating recombinant vectors, and the generation of HSV vectors is significantly more difficult and time-consuming than adenoviral or retroviral vector generation. Another disadvantage is that HSV is associated with transient gene expression and cytotoxicity. The use of HSV vectors has been reported in various in vivo applications including direct intraarticular injection,18 leading to efficient transduction of synovium.
Retroviral Vectors
Retroviruses are enveloped single-stranded RNA viruses that replicate through a double-stranded DNA intermediate. By integrating into the host DNA, the viral genomes are maintained for the life of the cell and are passed on to all daughter cells after cell division.
Moloney Murine Leukemia Viral Vectors
The more common retrovirus systems are based on the Moloney murine leukemia virus (MoMLV), and have been used in a large number of gene therapy clinical trials.15 MoMLV has been characterized extensively and has several attributes that make it attractive as a gene therapy vector: (a) the virus has no homology with human retroviruses, eliminating the possibility of recombination between the vector and resident human proviruses; (b) murine leukemia virus is nonpathogenic in humans; (c) the genome of the virus is relatively simple and amenable to molecular manipulation; (d) production is straightforward with moderately high titers; and (e) no viral proteins are contained in the vector, so infected cells are not antigenic. However, MoMLV requires division of the target cell for efficient transduction. MoMLV-based vectors can insert themselves randomly into the host DNA, resulting in stable modification of target cells, but also incurring the risk of insertional mutagenesis and activating a cell protooncogene or disrupting a tumor suppressor gene.13 The safety of such vectors has recently been called into question because children in a clinical protocol receiving these vectors have developed leukemia.15
Lentiviral Vectors
Lentiviruses are a subclass of retroviruses that can infect both dividing and nondividing cells. Like other retroviral vectors, they can be engineered to express no viral proteins, so that transduced cells are not antigenic. They hold up to 8 kb of foreign genetic material. Lentiviruses are genetically more complex than murine leukemia-based retroviruses, which makes molecular manipulation of the lentiviral genome more difficult. But the powerful attributes of infecting slow or nondividing cells and the maintenance of the vector genome in dividing cell populations make these vectors appealing tools for certain orthopaedic gene transfer applications.19 However, issues regarding their clinical applicability remain in question, due to the renewed fears about insertional mutagenesis.15
Vector Development Perspectives
It should be noted that vectorology is a rapidly developing field, and there have been significant efforts in the last few years to increase the safety and efficacy of the current viral vector systems. One area of interest involves the manipulation of promoters that drive transgene expression. To date, most investigations of gene transfer to cartilage have used vector systems with strong, viral promoters that provide constitutively high levels of transgene expression. This approach is useful during feasibility studies, where very high levels of transgene expression are used to overtly demonstrate the biologic effects that may be achieved with a particular gene and gene delivery method. However, for cartilage repair the stimulation of faithful synthesis of the complex architecture of articular cartilage, followed by its long-term maintenance, may require the use of more sophisticated types of tissue-specific regulatory elements.20 Unfortunately, the promoter regions for most chondrogenic genes are large, not well characterized, and consequently not routinely used in gene therapy. But as our knowledge about the native promoter systems of specific cell types expands, we may be able to harness tissue-specific promoters for a local and endogenous regulated gene expression.
Another method of achieving regulated transgene expression is the use of extrinsically regulated promoters. For example, a promoter that is activated only in the presence of tetracycline has been developed,21 and may be useful to turn off expression of the inserted gene after the therapeutic effect has been obtained.
Increasing the efficiency with which viral vectors infect specific cell populations may also increase the safety of gene therapy by allowing lower viral loads to be administered. Modifications on the vector capsid (including pseudotyping or molecular adaptors) to alter vector tropism away from its primary receptor is called “transductional targeting.” It is an important focus of vector development, addressing the problem of nonspecific or inefficient uptake. In the field of musculoskeletal tissue repair, being able to target a specific cell type may prove beneficial for transduction of cell types that would otherwise not be amenable, and may also limit the transgene expression to selected tissues of interest. On the other hand, as there are mixed populations of cell types in nearly all musculoskeletal repair tissues, it might not be necessary to target one specific cell type to facilitate repair. In the quest for better vectors, many researchers are attempting to combine the best features of different viruses in so-called hybrid or chimeric vectors, and there are many combinations of different beneficial vector features theoretically possible.
Nonviral Vector Systems for Orthopaedic Applications
In addition to viral vectors, several nonviral vectors (summarized in Table 26–3) have been used to transfer genes to joint tissues. Although certain cells such as myoblasts are able to take up naked DNA with some efficiency, complexion of DNA to different chemical formulations or to receptor/ligands increases DNA uptake and subsequent gene expression. Anionic and cationic liposomes are phospholipid vesicles that can fuse with cell membranes, and thus be used as gene transfer vehicles. Liposomes have been used to transfect chondrocytes in vitro, and also in various in vivo approaches for orthopaedic applications.22 Additional nonviral methods of introducing genetic material into cells include direct injection of plasmid DNA, or by firing DNA-coated microprojectiles through the cell membrane (biolistics), electroporation, and calcium phosphate precipitation. Although these physical gene transfer methods are easy to use, the transfection efficiencies are low, and generally have not been proven to work sufficiently in vivo.
Choosing a Gene-Delivery Approach and a Target Cell
There are two general methods for delivering a therapeutic gene into a target cell: a direct in vivo approach, and an indirect ex vivo approach. The direct approach involves the application of the vector directly into the target tissue of the subject, whereas the indirect approach involves the genetic modification of cells outside the body, followed by transplantation of the modified cells into the host. The choice of which gene transfer method to use is based on numerous considerations, including the gene to be delivered, the disease to be treated, and the vector used. In general, adenovirus, HSV, AAV vectors, lentivirus, and nonviral vectors may be used for in vivo and ex vivo delivery. Retroviral vectors, because of their inability to infect nondividing cells, are more suited for ex vivo use. Ex vivo approaches are generally more invasive, expensive, and technically tedious. However, they permit control of the transduced cells and safety testing prior to reimplantation. In vivo approaches are simpler, cheaper, and less invasive, but viruses are introduced directly into the body, which limits safety testing.
Toward the treatment and repair of damaged articular cartilage, the three primary candidate cell types to target genetic modification are synovial lining cells, chondrocytes, and mesenchymal stem cells.
Intraarticular Injection
Direct intraarticular injection of a vector or genetically modified cells into the joint space is, at least conceptually, the simplest approach to gene transfer to joint tissues. Following delivery, the vector or cells are distributed throughout the joint space to interact with all available receptive cells and surfaces. Because the synovium lines all the internal surfaces of the joint space, except for cartilage, and is highly cellular, it is the primary site of vector interaction. Direct intraarticular injection of vector or modified cells results in synthesis and release of therapeutic proteins into the joint space, which then bathe all available tissues, including cartilage. Considerable progress has been made toward defining the parameters critical to effective gene transfer to synovium and prolonged intraarticular expression. Recent data suggest that the use of immunologically compatible vector systems and homologous transgenes allows sustained intraarticular transgene expression.23 The effectiveness of synovial gene transfer of various transgenes is well documented in research directed toward rheumatoid arthritis,8 and the safety of indirect IL-1Ra gene transfer via a retroviral vector has been demonstrated successfully in a human phase I clinical trial.24 Data are beginning to emerge on its potential for treating OA with, for example, encouraging results for adenovirally delivered IL-1Ra to the joints of horses with experimental OA.25
There is a growing body of literature indicating that delivery of transgenes to synovium may not be compatible with many of the pleiotropic protein products considered for cartilage repair and regeneration. Gene transfer of TGF-β1 or BMP-2 to the synovium, for example, has been reported to cause severe joint fibrosis, swelling, and osteophyte and ectopic cartilage formation.26,27 This suggests caution when considering cDNAs for use in synovial gene transfer. Those selected should be safe when large amounts of gene product are expressed. Many antiinflammatory proteins have this property (see Table 26–1).
Gene Delivery to Cartilage Defects
For the gene-based delivery of certain factors to cartilage defects, a strategy whereby the transgenes are localized, and the gene products remain contained within the cartilage lesion, appears to be crucial. Possibly, the most direct manner by which to achieve this goal is by implantation of a three-dimensional matrix preloaded with a gene delivery vehicle into a defect (Fig. 26–2A). Such a device would localize the vector to the lesion while forming a support structure for the attachment of infiltrating cells. Within the matrix, cells would acquire the vector and locally secrete the stimulating transgene products. Such matrix-associated gene transfer approaches have been explored to augment bone healing by delivery of plasmid DNA encoding BMP-4.28 Recently, it was shown that collagen-glycosaminoglycan matrices incorporating plasmid-DNA at neutral pH were capable of transfecting seeded chondrocytes in culture, and enabling expression of a reporter gene for at least 28 days.29 Similarly, hydrated collagen-glycosaminoglycan matrices containing adenoviral vectors have been found to promote localized transgene expression in vivo following implantation into osteochondral defects in rabbit knees.30 In this system, bone marrow cells from the defect were infected by the virus within the matrix, and expressed the transgene locally for at least 21 days.30
Although transfer and expression of reporter genes has been observed by direct vector delivery to osteochondral lesions, it is not yet known whether this type of approach is capable of stimulating a sufficient biologic response for repair. One step toward a more cellular graft would be to supplement the vector-laden matrix with autologous cells that are intraoperatively readily available. Cells from bone marrow aspirates [bone marrow cells (BMCs)] fulfill this requirement and can be used in an abbreviated, genetically enhanced tissue engineering approach (Fig. 26–2B). The advantage of direct, in vivo delivery systems of this type is that they can be conducted within one surgical setting, thus saving time and cost, while avoiding labor intensive ex vivo culture of cells. Their limitation, however, is the lack of control over gene transfer following implantation.
Gene transfer is also being explored as a mechanism for enhancing ex vivo cell delivery approaches for cartilage repair (Fig. 26–2C). Although laborious and expensive, this indirect approach has several advantages: (a) a pure population of cells can be selected under controlled conditions; (b) it provides a highly cellular, space-filling entity; (c) it localizes transgene expression to the site of injury; (d) no free vector particles are administered to the subject; and (e) safety testing prior to reimplantation is possible. Various studies have been conducted to explore gene transfer to chondrocytes and mesenchymal progenitor cells to enhance ex vivo cell delivery approaches for cartilage repair.
Gene Transfer to Chondrocytes
Chondrocytes in monolayers are readily amenable to gene transfer by viral vectors such as MoMLV, lentivirus, adenovirus, and AAV. Adenoviral-mediated delivery of cDNAs for TGF-β1 BMP-2, IGF-I, or BMP-7 has been shown to stimulate the expression of a cartilage-specific ECM, with increased proteoglycan and type II collagen production and a decreased tendency toward dedifferentiation.31–35 Although chondrocytes have been somewhat resistant to transfection with plasmid DNA, formulations with certain commercially available lipid-based reagents such as FuGENE6 and Lipofectin have been found to enhance the efficiency of DNA uptake, especially if the surrounding matrix is subjected to mild enzymatic digestion.22 Viral-based vectors, however, are capable of generating far higher levels of transgene expression with greater persistence.
Having shown the capacity of gene transfer approaches to beneficially alter the molecular properties of articular chondrocytes, the research focus has shifted toward exploring methods of delivery to cartilage defects. One approach would be to genetically augment the autologous chondrocyte implantation (ACI) procedure, with the given advantage that ACI is already clinically established and the associated infrastructure for its application is already in place. There is an accumulating body of literature that shows that genetically altered chondrocytes retain the ability to attach to and colonize cartilage explants in culture, and are capable of expressing transgene products at functional levels following engraftment in vivo. Transplantation of transfected IGF-I expressing chondrocytes led to significant resurfacing and thicker tissue enriched with proteoglycans and type II collagen, compared with transplanted control cells.22 In addition, adenoviral-mediated IL-1Ra gene transfer to chondrocytes resulted in resistance to IL-1—induced proteoglycan degradation after engraftment.36
FIGURE 26–2 Gene delivery approaches for the treatment of cartilage defects. (A) Matrix-associated in vivo gene transfer. In vivo gene transfer involves incorporation of the gene transfer vector into a biologically compatible matrix, which is implanted within an osteochondral defect. Bone marrow cells (BMCs) migrate into the matrix, encounter the vector, and acquire the desired gene. Genetically modified cells locally express and secrete the transgene products that influence mesenchymal progenitors toward chondrogenic pathways. This procedure does not require ex vivo cell manipulation. (B) Abbreviated ex vivo genetically enhanced tissue engineering. To facilitate this direct approach, the incorporation of a vector into the matrix can be conducted together with the incorporation of cells that could be harvested a single operative setting (like BMCs from bone marrow aspirates). (C) Traditional ex vivo genetically enhanced tissue engineering. For ex vivo gene transfer, target cells (e.g., chondrocytes or mesenchymal stem cells) are harvested in an initial surgical procedure. Then they are incubated with the vector of interest, selected, and amplified. The genetically modified cells are then seeded into a biologic matrix, which can be further cultured under controlled conditions. In a second surgical procedure the constructs are implanted into a cartilage lesion. This procedure may permit transgene expression to be concentrated within the cell types responsible for generating the repair tissue. In both approaches (B and C), locally secreted proteins could influence cartilage regeneration by implanted cells as well as those that may migrate into the defect after implantation of the matrix.
As an alternative to delivery in suspension, efforts have also been made to augment tissue engineering procedures using genetically modified chondrocytes (Fig. 26–2C). For this, the cells are transduced/transfected in monolayer and then seeded into a matrix for subsequent implantation into chondral or osteochondral defects.37
Initial studies demonstrated that chondrocytes transduced with various vectors encoding marker genes, when embedded in collagenous matrices38–40 or alginate microspheres41 and delivered to osteochondral defects in rabbits, could support prolonged transgene expression for several weeks.
Results of efficacy studies are just beginning to emerge, showing the effects of genetically modified chondrocytes in cartilage lesions in vivo. In ex vivo approaches, adenovirally transduced chondrocytes expressing BMP-7,42 incorporated in a matrix of autogenous fibrin, were implanted into full-thickness articular cartilage defects in horses. Four weeks after surgery, an increased tissue volume and accelerated formation of a proteoglycan and type II collagen—rich matrix could be observed in the BMP-7—treated defects compared with control defects treated with irrelevant marker genes. However, after 8 months, the levels of type II collagen and proteoglycan, and the mechanical characteristics of the treated defects compared with the controls were similar. This was attributed in part to the declining number of allografted chondrocytes that persisted in the defects after 8 months.42 It is encouraging, however, that genetically modified chondrocytes can be used to augment a cartilage repair process in a large animal model.
Gene Transfer to Chondroprogenitor Cells
Major limitations for the use of autologous chondrocytes for cartilage repair are that they require the surgical removal of non—weight-bearing articular cartilage as a source of cells, of which there is a very limited supply, and that chondrocytes dedifferentiate during expansion with a subsequent loss of the chondrocytic phenotype. An alternative source of cells that can be harnessed for cartilage repair procedures are mesenchymal progenitor cells, also referred to as mesenchymal stem cells (MSCs). These cells have the capacity to differentiate into the various mesenchymal tissues including cartilage, bone, muscle, fat, and others.43 MSCs have been isolated from several sources, including bone marrow,44 bone chips,45 adipose tissue,46 periosteum, and perichondrium. MSCs have been shown to maintain their multilineage potential with passage in culture,47 making them an attractive cell source for the repair of full-thickness osteochondral defects.
MSCs appear to be reasonably receptive to transduction with recombinant adenoviral vectors, retrovirus, lentivirus, and AAV27,43,48,49 (and unpublished observations). Various in vitro systems have established that mesenchymal progenitor cells undergo chondrogenesis under certain three-dimensional culture conditions, provided that certain chondrogenic factors like TGF-β1 or BMP-2 are present.47,50,51 Following plasmid-mediated BMP-2 and BMP-452,53 and retrovirus-mediated BMP-254 gene transfer, the murine mesenchymal progenitor cell line C3H10T1/2 was able to differentiate along the chondrogenic lineage. Primary mesenchymal progenitor cells, genetically modified to express TGF-β1 or BMP-248 were also found to undergo chondrogenesis in aggregate culture.
Initial studies have been conducted to apply gene transfer technologies to MSCs in vivo. One approach focuses on the delivery of genetically modified MSCs through tissue engineering methods (Fig. 26–2C). In one study, retrovirally transduced periosteal cells expressing BMP-7, following selection and expansion, were seeded into polyglycolic acid scaffolds and implanted into rabbit osteochondral defects. Relative to control groups, the defects treated with the BMP-7 modified progenitors showed improved regeneration of bone and cartilage at 8 to 12 weeks postimplantation.55,56 Using the same experimental approach, the delivery of sonic hedgehog (SHH) cDNA was recently found to result in superior overall repair after 12 weeks compared with BMP-7—treated defects, and both were superior to controls.57 In another ex vivo approach, adenoviral-mediated BMP-2 and IGF-I gene transfer to mesenchymal progenitor cells from rib perichondrium was accomplished for the repair of partial-thickness lesions in rats. This resulted in a type II collagen and proteoglycan-rich repair tissue, compared with the fibrous tissue of the control defects after 8 weeks.27
In an effort to simplify these kinds of time-consuming and expensive ex vivo procedures, we have recently begun to explore methods for direct, in vivo gene transfer to sites of cartilage damage. In a matrix-associated gene transfer approach (Fig. 26–2A) to rabbit osteochondral defects, hydrated collagen-glycosaminoglycan matrices preloaded with adenoviral vectors have been found to promote localized expression of marker genes for at least 3 weeks by bone marrow cells that migrated into the matrix and became transduced.30 In an attempt to take this approach a step further, we tried to find ways to facilitate this gene transfer approach with an endogenous cellular and space-filling entity (Fig. 26–2B). Thus, in an abbreviated, genetically enhanced tissue engineering approach, we demonstrated that when fresh bone marrow aspirates were mixed with a solution of recombinant adenoviral vectors and allowed to coagulate, MSCs within the coagulum acquired and expressed the transgene for several weeks after implantation into osteochondral defects in rabbits.30 Studies are underway to investigate how these advances can be harnessed to achieve cartilage repair.
Conclusions and Future Challenges
Gene transfer techniques are very powerful tools that might help us to overcome the limitations of the current treatments for damaged articular cartilage. This chapter summarized the progress toward the development of gene-based strategies for articular cartilage repair. It has been shown that exogenous genes can be delivered to cartilaginous defects by several methods, and that the corresponding transgenes can be expressed for extended periods of time. Nonetheless, what remains to be determined are the level and duration of gene expression needed, and the gene or combination of genes necessary to meet the complexities of treating this tissue. The results of recent efficacy studies provide evidence that vector-mediated delivery of certain growth factors can be used to elicit favorable biologic responses in vivo, and as more data surface, a clearer picture of the functional boundaries of these applications and the parameters critical for success will appear. However, much work remains to be done to establish the appropriate combination of cells, genes, and delivery methods for achieving long-term repair of articular cartilage.
The use of gene transfer techniques to facilitate musculoskeletal tissue repair offers what is perhaps an immediate opportunity for a clinical application of gene therapy. Compared with the treatment of genetic diseases, where lifelong expression of a corrective transgene is required, or cancer, where extraordinarily high efficiency and specificity of transgene delivery is required to eradicate tumor cells from the body, tissue repair may only require transient, localized expression of a specific transgene product. The use of integrating vector systems and lifelong expression of potent transgenes might thus not be necessary for most applications, and their associated risks could be avoided. Furthermore, because current medical and surgical procedures have limited effectiveness, a clinically useful gene-based repair system need not necessarily demonstrate complete regeneration of normal tissue. Because surgical procedures for cartilage repair are almost always elective, and cartilage injuries are not life-threatening, the safety of gene transfer approaches for repair is of particular importance.
REFERENCES
1. Buckwalter JA. Articular cartilage injuries. Clin Orthop 2002;402:21–37
2. Evans CH, Ghivizzani SC, Smith P, Shuler FD, Mi Z, Robbins PD. Using gene therapy to protect and restore cartilage. Clin Orthop 2000;379(suppl):S214–S219
3. Hickey DG, Frenkel SR, Di Cesare PE. Clinical applications of growth factors for articular cartilage repair. Am J Orthop 2003;32:70–76
4. Vortkamp A. Interaction of growth factors regulating chondrocyte differentiation in the developing embryo. Osteoarthritis Cartilage 2001;9(suppl A):S109–S117
5. Lefebvre V, Behringer RR, de Crombrugghe B. L-Sox5, Sox6 and Sox9 control essential steps of the chondrocyte differentiation pathway. Osteoarthritis Cartilage 2001;9(suppl A):S69–S75
6. Hoffmann A, Gross G. BMP signaling pathways in cartilage and bone formation. Crit Rev Eukaryot Gene Expr 2001;11:23–45
7. Dharmavaram RM, Liu G, Tuan RS, Stokes DG,Jimenez SA. Stable transfection of human fetal chondrocytes with a type II procollagen minigene: expression of the mutant protein and alterations in the structure of the extracellular matrix in vitro. Arthritis Rheum 1999;42:1433–1442
8. Robbins PD, Evans CH, Chernajovsky Y. Gene therapy for arthritis. Gene Ther 2003;10:902–911
9. Sullenger BA, Gilboa E. Emerging clinical applications of RNA. Nature 2002;418:252–258
10. Crooke ST. Molecular mechanisms of action of antisense drugs. Biochim Biophys Acta 1999;1489:31–44
11. Tomita N, Morishita R, Tomita T, Ogihara T. Potential therapeutic applications of decoy oligonucleotides. Curr Opin Mol Ther 2002;4:166–170
12. McManus MT, Sharp PA. Gene silencing in mammals by small interfering RNAs. Nat Rev Genet 2002;3:737–747
13. Oligino TJ, Yao Q, Ghivizzani SC, Robbins P. Vector systems for gene transfer to joints. Clin Orthop 2000;379(suppl):S17–S30
14. Hardy S, Kitamura M, Harris-Stansil T, Dai Y, Phipps ML. Construction of adenovirus vectors through Cre-lox recombination. J Virol 1997;71:1842–1849
15. Thomas CE, Ehrhardt A, Kay MA. Progress and problems with the use of viral vectors for gene therapy. Nat Rev Genet 2003;4:346–358
16. McCarty DM, Monahan PE, Samulski RJ. Self-complementary recombinant adeno-associated virus (scAAV) vectors promote efficient transduction independently of DNA synthesis. Gene Ther 2001;8:1248–1254
17. Madry H, Cucchiarini M, Terwilliger EF, Trippel SB. Recombinant adeno-associated virus vectors efficiently and persistently transduce chondrocytes in normal and osteoarthritic human articular cartilage. Hum Gene Ther 2003;14:393–402
18. Oligino T, Ghivizzani S, Wolfe D, et al. Intra-articular delivery of a herpes simplex virus IL-1Ra gene vector reduces inflammation in a rabbit model of arthritis. Gene Ther 1999;6:1713–1720
19. Gouze E, Pawliuk R, Pilapil C, et al. In vivo gene delivery to synovium by lentiviral vectors. Mol Ther 2002;5:397–404
20. Stein GS, Lian JB, Stein JL, van Wijnen AJ. Bone tissue specific transcriptional control: options for targeting gene therapy to the skeleton. Cancer 2000;88(suppl):2899–2902
21. Gossen M, Bujard H. Tight control of gene expression in mammalian cells by tetracycline-responsive promoters. Proc Natl Acad Sci U S A 1992;89:5547–5551
22. Madry H, Trippel SB. Efficient lipid-mediated gene transfer to articular chondrocytes. Gene Ther 2000;7:286–291
23. Gouze E, Pawliuk R, Gouze JN, et al. Lentiviral-mediated gene delivery to synovium: potent intra-articular expression with amplification by inflammation. Mol Ther 2003;7:460–466
24. Evans CH, Robbins PD, Ghivizzani SC, et al. Clinical trial to assess the safety, feasibility, and efficacy of transferring a potentially antiarthritic cytokine gene to human joints with rheumatoid arthritis. Hum Gene Ther 1996;7:1261–1280
25. Frisbie DD, Ghivizzani SC, Robbins PD, Evans CH, McIlwraith CW. Treatment of experimental equine osteoarthritis by in vivo delivery of the equine interleukin-1 receptor antagonist gene. Gene Ther 2002;9:12–20
26. Mi Z, Ghivizzani SC, Lechman E, Glorioso JC, Evans CH, Robbins PD. Adverse effects of adenovirus-mediated gene transfer of human transforming growth factor beta 1 into rabbit knees. Arthritis Res Ther 2003;5:R132–R139
27. Gelse K, von der Mark K, Aigner T, Park J, Schneider H. Articular cartilage repair by gene therapy using growth factor-producing mesenchymal cells. Arthritis Rheum 2003;48:430–441
28. Bonadio J, Smiley E, Patil P, Goldstein S. Localized, direct plasmid gene delivery in vivo: prolonged therapy results in reproducible tissue regeneration [see comments] Nat Med 1999;5:753–759
29. Samuel RE, Lee CR, Ghivizzani SC, et al. Delivery of plasmid DNA to articular chondrocytes via novel collagen-glycosaminoglycan matrices. Hum Gene Ther 2002;13:791–802
30. Pascher A, Palmer G, Steinert A, et al. Gene delivery to cartilage defects using coagulated bone marrow aspirate. Gene Ther 2004;11:133–141
31. Hidaka C, Quitoriano M, Warren RF, Crystal RG. Enhanced matrix synthesis and in vitro formation of cartilage-like tissue by genetically modified chondrocytes expressing BMP-7. J Orthop Res 2001;19:751–758
32. Brower-Toland BD, Saxer RA, Goodrich LR, et al. Direct adenovirus-mediated insulin-like growth factor I gene transfer enhances transplant chondrocyte function. Hum Gene Ther 2001;12:117–129
33. Nixon AJ, Fortier LA, Williams J, Mohammed H. Enhanced repair of extensive articular defects by insulin-like growth factor-I-laden fibrin composites. J Orthop Res 1999;17:475–487
34. Shuler FD, Georgescu HI, Niyibizi C, et al. Increased matrix synthesis following adenoviral transfer of a transforming growth factor beta1 gene into articular chondrocytes [In Process Citation]. J Orthop Res 2000;18:585–592
35. Smith P, Shuler FD, Georgescu HI, et al. Genetic enhancement of matrix synthesis by articular chondrocytes: comparison of different growth factor genes in the presence and absence of interleukin-1. Arthritis Rheum 2000;43:1156–1164
36. Baragi VM, Renkiewicz RR, Jordan H, Bonadio J, Hartman JW, Roessler BJ. Transplantation of transduced chondrocytes protects articular cartilage from interleukin 1-induced extracellular matrix degradation. J Clin Invest 1995;96:2454–2460
37. Kaps C, Bramlage C, Smolian H, et al. Bone morphogenetic proteins promote cartilage differentiation and protect engineered artificial cartilage from fibroblast invasion and destruction. Arthritis Rheum 2002;46:149–162
38. Ikeda T, Kubo T, Arai Y, et al. Adenovirus mediated gene delivery to the joints of guinea pigs. J Rheumatol 1998;25:1666–1673
39. Kang R, Marui T, Ghivizzani SC, et al. Ex vivo gene transfer to chondrocytes in full-thickness articular cartilage defects: a feasibility study. Osteoarthritis Cartilage 1997;5:139–143
40. Baragi VM, Renkiewicz RR, Qiu L, et al. Transplantation of adenovirally transduced allogeneic chondrocytes into articular cartilage defects in vivo. Osteoarthritis Cartilage 1997;5:275–282
41. Madry H, Cucchiarini M, Stein U, et al. Sustained transgene expression in cartilage defects in vivo after transplantation of articular chondrocytes modified by lipid-mediated gene transfer in a gel suspension delivery system. J Gene Med 2003;5:502–509
42. Hidaka C, Goodrich LR, Chen CT, Warren RF, Crystal RG, Nixon AJ. Acceleration of cartilage repair by genetically modified chondrocytes over expressing bone morphogenetic protein-7. J Orthop Res 2003;21:573–583
43. Caplan AI. Mesenchymal stem cells and gene therapy. Clin Orthop 2000;379(suppl):S67–S70
44. Prockop DJ. Marrow stromal cells as stem cells for nonhematopoietic tissues. Science 1997;276:71–74
45. Noth U, Osyczka AM, Tuli R, Hickok NJ, Danielson KG, Tuan RS. Multilineage mesenchymal differentiation potential of human trabecular bone-derived cells. J Orthop Res 2002;20:1060–1069
46. Zuk PA, Zhu M, Mizuno H, et al. Multilineage cells from human adipose tissue: implications for cell-based therapies. Tissue Eng 2001;7:211–228
47. Yoo JU, Barthel TS, Nishimura K, et al. The chondrogenic potential of human bone-marrow-derived mesenchymal progenitor cells. J Bone Joint Surg Am 1998;80:1745–1757
48. Yoo JU, Mandell I, Angele P, Johnstone B. Chondrogenitor cells and gene therapy. Clin Orthop 2000;379(suppl):S164–S170
49. Mosca JD, Hendricks JK, Buyaner D, et al. Mesenchymal stem cells as vehicles for gene delivery. Clin Orthop 2000;379(suppl):S71–S90
50. Haas AR, Tuan RS. Chondrogenic differentiation of murine C3H10T1/2 multipotential mesenchymal cells: II. Stimulation by bone morphogenetic protein-2 requires modulation of N-cadherin expression and function. Differentiation 1999;64:77–89
51. Johnstone B, Hering TM, Caplan AI, Goldberg VM, Yoo JU. In vitro chondrogenesis of bone marrow-derived mesenchymal progenitor cells. Exp Cell Res 1998;238:265–272
52. Steinert A, Weber M, Dimmler A, et al. Chondrogenic differentiation of mesenchymal progenitor cells encapsulated in ultrahigh-viscosity alginate. J Orthop Res 2003;21:1090–1097
53. Ahrens M, Ankenbauer T, Schroder D, Hollnagel A, Mayer H, Gross G. Expression of human bone morphogenetic proteins-2 or -4 in murine mesenchymal progenitor C3H10T1/2 cells induces differentiation into distinct mesenchymal cell lineages. DNA Cell Biol 1993;12:871–880
54. Carlberg AL, Pucci B, Rallapalli R, Tuan RS, Hall DJ. Efficient chondrogenic differentiation of mesenchymal cells in micromass culture by retroviral gene transfer of BMP-2. Differentiation 2001;67:128–138
55. Mason JM, Grande DA, Barcia M, Grant R, Pergolizzi RG, Breitbart AS. Expression of human bone morphogenic protein 7 in primary rabbit periosteal cells: potential utility in gene therapy for osteochondral repair. Gene Ther 1998;5:1098–1104
56. Mason JM, Breitbart AS, Barcia M, Porti D, Pergolizzi RG, Grande DA. Cartilage and bone regeneration using gene-enhanced tissue engineering. Clin Orthop 2000;379(suppl):S171–S178
57. Grande DA, Mason J, Light E, Dines D. Stem cells as platforms for delivery of genes to enhance cartilage repair. J Bone Joint Surg Am 2003;85-A(suppl 2):111–116
< div class='tao-gold-member'>
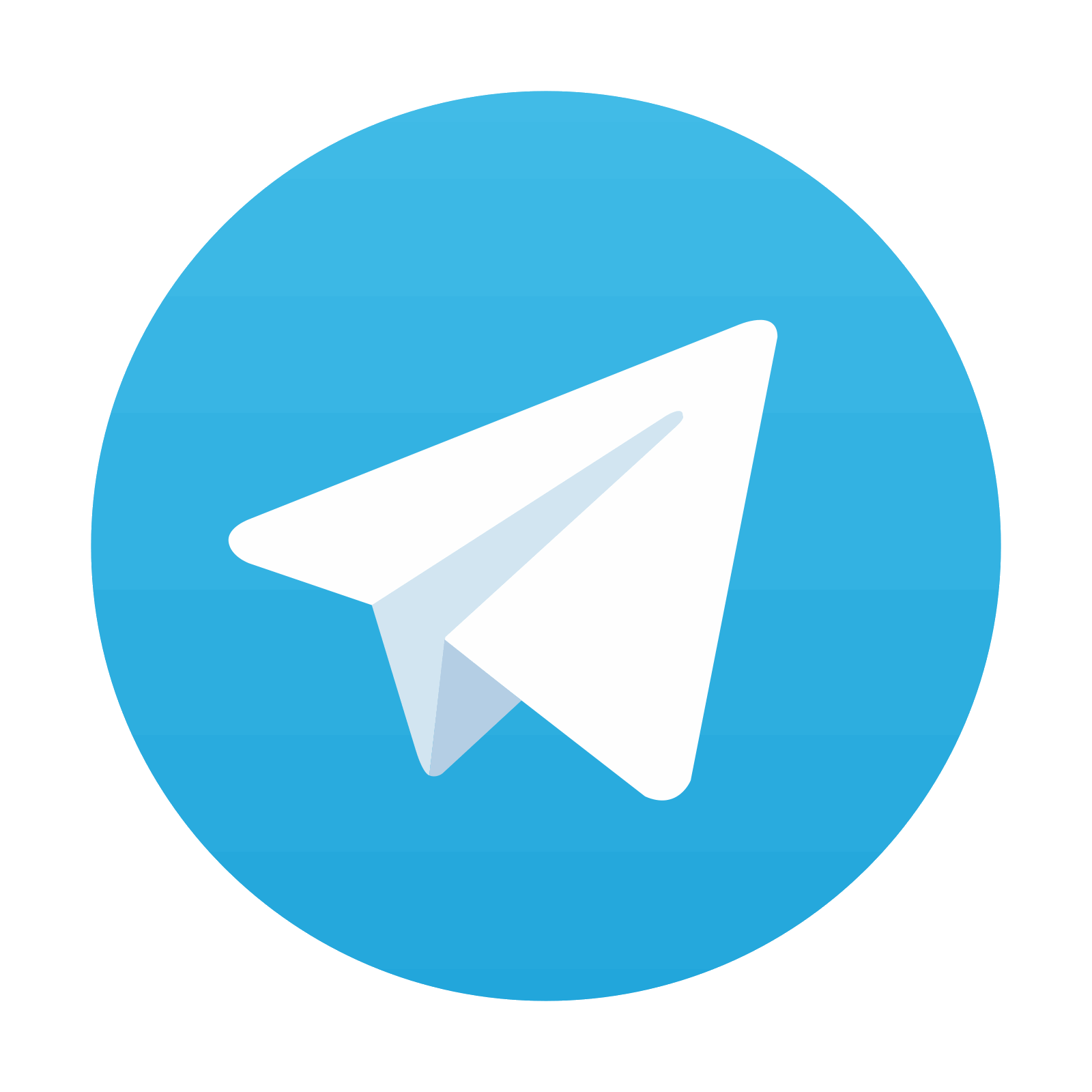