Gait and Gait Disturbances
Thomas P. Andriacchi, PhD
Jennifer C. Erhart-Hledik, PhD
Dr. Erhart-Hledik or an immediate family member serves as a paid consultant to or is an employee of HingeHealth and Moximed. Neither Dr. Andriacchi nor any immediate family member has received anything of value from or has stock or stock options held in a commercial company or institution related directly or indirectly to the subject of this chapter.
Keywords: Clinical applications of gait analysis; gait disturbances; gait measures
INTRODUCTION
The purpose of this chapter is to highlight new evidence that shows specific elements of gait can be used to assess functional health, the state of many pathological conditions, and the risk of progression of pathological conditions and provide objective assessment of treatment outcome. This information is particularly important for orthopedic practice because one of the primary goals of orthopedic treatment is restoration of function.
The field of gait analysis continues to evolve producing clinically relevant new information. Section 2 provides definitions and methods for interpreting gait measures that can be considered signals for both musculoskeletal pathology as well as tissue adaptation. Section 3 illustrates how musculoskeletal pathology provides a signaling pathway for gait disturbances that reflect the underlying pathology. Conversely, Section 4 will illustrate how specific features of gait mechanics can stimulate a signaling pathway where tissue structure and biology adapt to gait mechanics. Both signaling pathways suggest there are interactions between the elements of gait mechanics, biology, and structure that offer new opportunities to address the multifaceted complexity of musculoskeletal pathology.
GAIT MEASURES DEFINITION AND INTERPRETATION
Time-distance measures offer an assessment of the overall pattern of gait that can provide an objective test for gait disturbances associated with pathology and assess treatment outcomes. These measures are among the easiest gait measures to capture1 and are usually quantified by walking speed (average speed of forward progression in meters/second), stride length (distance between ipsilateral heel strikes in meters), and cadence (steps per unit time in step count/minute). In some cases, stride length can be normalized to height or lower extremity length to allow for comparisons among individuals with differences in statures.
It is important to note that time-distance measures are interrelated with strong associations between metrics such as stride length and walking speed. For example, a healthy subject has a shorter stride length when walking slower, and stride length linearly increases with walking speed (Figure 1). The fact that gait metrics like stride length are dependent on walking speed is critical to the clinical application of gait analysis because patients with gait disturbances often walk slower than healthy subjects (Figure 1). Thus, gait metric comparisons between healthy subjects and patients should account for the influence of walking speed.2
The fact that gait measures are reproducible is another important consideration in the clinical application of gait analysis as illustrated in Figure 1. A healthy subject tested on two occasions will have the same relationship between stride length and walking speed. The reproducibility of gait mechanics for healthy subjects is governed by minimizing metabolic energy consumption3,4 that permits walking efficiently for long distances. Thus, any changes in the stride length-walking speed relationship would suggest a change in the health status of the subject. The clinical application of time-distance measures will be discussed further in the Clinical Applications section.
Kinematics measures during gait provide more specific information than time-distance measures which can be related to the motion of individual joints. Joint kinematics is normally described in terms of relative angles between adjacent limb segments. In practice, human gait5 is often described in terms of the sagittal plane motion (eg, flexion-extension at the hip and knee as well as dorsiflexion-plantar flexion at the ankle). The patterns of flexion-extension motion are reproducible among the normal population. As illustrated for healthy gait (Figure 2): hip motion smoothly cycles from a peak flexion at heel strike (˜20°) to peak extension prior to toe off to reaching a peak in flexion during swing phase; knee motion cycles from extension at heel strike, to local maximum flexion (˜20°) during midstance phase (load response), toward extension in late stance (terminal extension) and flexes again prior to swing phase where the knee flexes to approximately 60°; ankle motion cycles from plantar flexion in early stance phase to dorsiflexion in late stance phase and reverses to plantar flexion during swing phase returning to a neutral position prior to heel strike. The smooth patterns of motions illustrated in Figure 2 are consistent with walking with an energy-efficient gait that allows walking long distances with minimal fatigue. In addition, these motion patterns allow for some of the impact load to be absorbed by soft tissues and reduce impact directly on the joint surfaces. For example, knee flexion during midstance (Figure 2), commonly called load response, allows for some of the downward load in early stance to be absorbed through the extensor mechanism rather than through the cartilage at the knee.
The smooth patterns of motion during healthy gait can substantially change with musculoskeletal pathology because gait patterns will be reprogrammed to adapt to conditions such as pain, instability, or disruption of normal muscle activation. As such, gait disturbances typically can cause untimely fatigue during walking as the pattern of gait is no longer programmed to optimize energy consumption but rather to adapt to the specific nature of the pathological condition.
Kinetic measurements provide clinically important measures that can be related to the loading on the musculoskeletal system. Commonly, musculoskeletal loading during gait is described in terms of forces and moments. As will be illustrated in this chapter, gait analysis can provide a useful tool to noninvasively measure joint loading (forces and moments at the joints) during ambulation. At present, these kinetic measures have been primarily the domain of the research laboratory and have not migrated to routine clinical practice or thinking, yet an understanding of joint loading during gait can provide a deeper comprehension of the treatment of musculoskeletal injury and disease.
Given the clinical importance of understanding the nature of joint loading during gait, it is useful to explore some of the methods and assumptions used when capturing and interpreting kinetic measures. The fact that forces and moments are abstract constructs that cannot be seen often presents a barrier to clinical interpretation. Understanding a few general concepts (illustrated for the knee in Figure 3) will help facilitate clinical interpretation of kinetic measures. Most clinical gait studies are conducted noninvasively with external sensors. As such, direct gait loading measured in the laboratory is described as “external loading”. These external loading measures during walking include the external ground reaction and inertial forces/moments (due to mass, inertia, velocity, and acceleration) that act on each limb segment (Figure 3, A and D). These forces and moments can be resolved to the joint center6 and are described as external measures.
Clinical interpretation of the external measurement requires relating the external loads to internal loads7 generated by muscles and joint contact forces (Figure 3, B and E). For the knee joint example (Figure 3, A), the external flexion moment is important because this moment must be balanced by a force generated by the quadriceps muscle (Figure 3, B), and patterns of the flexion-extension moment can be interpreted as reflecting quadriceps function during walking. Again, using the knee example, the external adduction moment can be related to the internal joint reaction forces on the medial and lateral compartment of the knee and provides a basis for understanding why there are greater forces on the medial compartment of the knee relative to the lateral compartment.7
The moment magnitudes are dependent on walking speed, and comparisons between moment magnitudes should also account for walking speed. The moment magnitude is also dependent on weight and height (which influences lever arms, or the distance over which the force acts on the joint); thus, moments are often normalized by expressing the moment as a percentage of body weight X height to allow comparison between subjects of different stature. Finally, the interpretation of the external moment should include the possibility that the internal moment balance can be due to the summation of both antagonistic and synergistic muscle forces. For example, an external moment tending to flex the knee would be balanced by a “net” (extensors minus flexors) internal moment tending to extend the knee. In spite of the numerous factors that could influence the moment, these patterns are quite repeatable for healthy gait.8 The repeatability of these patterns is important because changes from these patterns can be used to identify gait disturbances that are associated with specific pathology. Some examples are given in the Clinical Applications section later in this chapter.
Reproducibility of gait measures is in general critical to the clinical application of gait. Specifically, gait patterns are governed by criteria that act at the interface between physics and human physiology in a manner that the mechanics of healthy gait are ruled by a tendency to optimize metabolic energy consumption. For example, self-selected walking speed (mechanical metric) for healthy subjects is directly associated with optimizing energy expenditure.3,4 As such, healthy gait patterns at self-selected speeds reflect the tendency to optimize the capacity to walk with minimal energy consumption and provide a basis for explaining why healthy gait patterns are highly repeatable.
A pathological condition that causes pain or instability or affects muscle activation or strength will cause the body to reprogram gait patterns based on the pathological condition. For example, a limp due to pain reflects a reprogramming of the pattern of locomotion (gait disturbance) that is governed by the pathological condition rather than optimizing energy consumption. Evidence2,5 that specific gait disturbances are also reproducible and can be related to the pathological condition when controlled for speed provides a basis for using gait to assess musculoskeletal pathology.
Another important consideration in utilizing gait measures is that the gait-testing modality should not encumber natural patterns of locomotion to avoid masking the nature of any adaptive gait disturbances that reflect adaptations to the underlying pathology. Many orthopedic conditions such as osteoarthritis are influenced by cyclic loading associated with the repeated patterns of movement during natural gait. Even testing methods such as walking on a treadmill can create gait changes relative to natural overground walking9 (eg, significant changes in muscle activation and joint moments) that can mask the true nature of a clinically relevant adaptive gait pattern. Thus, capturing the unencumbered characteristics of gait is important for evaluating musculoskeletal pathology.
Finally, it should be noted that the methods and principles summarized above for the knee can be applied to the hip and ankle where additional detail can be found in the literature.6,8
CLINICAL APPLICATIONS OF GAIT ANALYSIS
Certain types of musculoskeletal pathology provide signals to the locomotor system to modify patterns of locomotion (a gait disturbance) that reflect the presence and nature of the pathological condition. Gait disturbances can be positive (mitigate pathology) or negative (intensify pathology) depending on the individual patient’s capacity to respond to the pathological signaling.
Objective assessment of patients with total knee replacement (TKR) can be quite variable using common clinical methods based on patient-reported outcomes. However, it has been shown that objective assessment of functional improvement following TKR can be achieved using relatively inexpensive and efficient time-distance measures. The analysis was based on the fact that time-distance measures are highly reproducible (Figure 1) for healthy subjects and provides a useful basis for detecting general disturbances in gait patterns. Treatments, therefore, can be evaluated by testing changes in these measures over time. Given the walking speed dependency of gait measures, it is useful to test these measures as a function of walking speed by asking a subject to walk at their comfortable normal speed, slower than normal, and faster than normal. The resulting data will present a spread of measures over a range of walking speeds. The stride length-walking speed relationship (Figure 1) is linear over a range of speeds, and this relationship can be useful for making comparison to healthy subjects as well as comparing changes in patient gait patterns following treatment.
A study2 of patients at 3, 6, and 9 months following TKR surgery was conducted to test the length of time it took for
a patient to recover stable gait following surgery (Figure 1) relative to healthy gait patterns. TKR patients and healthy subjects were tested for differences in stride length over a range of speeds to address the walking speed influence. The results showed that the TKR patient walked slower than a healthy subject. Further, the TKR patient had a stride length-walking speed relationship that was different from healthy subjects at the three time points following surgery, and the patient did not return to normal gait at 9 months past surgery (Figure 1). However, the results did demonstrate that the gait of the TKR subject changed toward normal between 3 and 6 months past surgery but did not change between 6 and 9 months past surgery, suggesting that gait changes following TKR stabilize at 6 months past surgery.
a patient to recover stable gait following surgery (Figure 1) relative to healthy gait patterns. TKR patients and healthy subjects were tested for differences in stride length over a range of speeds to address the walking speed influence. The results showed that the TKR patient walked slower than a healthy subject. Further, the TKR patient had a stride length-walking speed relationship that was different from healthy subjects at the three time points following surgery, and the patient did not return to normal gait at 9 months past surgery (Figure 1). However, the results did demonstrate that the gait of the TKR subject changed toward normal between 3 and 6 months past surgery but did not change between 6 and 9 months past surgery, suggesting that gait changes following TKR stabilize at 6 months past surgery.
The above example illustrates that time-distance measurements can be useful for objective assessment of treatment outcome. Given that patient-reported outcomes alone can vary substantially among patients, the potential to have a simple metric that can objectively assess functional outcome could be important in evaluating the efficacy of procedures such as TKR. In addition, these measures can be obtained using simple sensors without the need of a full gait laboratory. Although useful, these measures cannot give the specific cause of the gait disturbance.
Hip osteoarthritis (OA) and femoral acetabular impingement (FAI) have common kinematic gait disturbances that provide insight into the pathomechanics of these conditions as well as a basis for evaluating treatment outcomes. Patients with hip OA were studied10 to test for gait characteristics that could be associated with motion of the hip during walking. Specifically, hip flexion-extension motion was studied in patients with unilateral OA of the hip and compared with a group of healthy subjects with a similar demographic distribution. The patients who had hip OA had a consistent gait disturbance that was characterized by a decreased dynamic range of motion of the hip and with a disturbance in the normal smooth pattern of hip flexion during gait. The disturbance was characterized by a hesitation or reversal in the direction of the sagittal plane motion as the hip extended in midstance (Figure 4). This reversal was documented in 74% of the OA patients and was evident in only 5% of the healthy subjects.10 The gait adaptations in patients who had hip OA were related to pain and limitations in passive motion. Many of the features of the adaptations were subtle and not detectable through visual observation alone. As such, the nature of the gait disturbance illustrated in Figure 4 provides an objective marker of the state of OA at the hip.
Interestingly, FAI patients were found to have a similar pattern of hip motion during gait to patients with hip OA.11 The presence of these reversals in FAI patients reinforces the idea of FAI being a precursor to hip OA. This observation is important because FAI was thought to be a risk factor for developing hip OA. Thus, an objective functional marker for the risk of hip OA was needed as clinical outcome measures are generally subjective or not conducted under actual activities of daily living.
The above observations were used in a subsequent study12 to evaluate the outcome of treatment for FAI. Specifically, the study12 demonstrated that the pattern of hip flexion-extension motion during walking returned to normal after arthroscopic surgery was performed to treat FAI. These findings suggest that walking mechanics can provide a useful objective measure to quantitatively assess the outcome of surgical intervention for FAI. The patterns of changes in motion with FAI provide insight into the functional pathologic abnormalities of the disease. The observed reduced hip flexion-extension motion preoperatively may be the result of an adaptive response to pain emanating from labral tears and chondral defects and/or mechanical abutment of the abnormal bony morphology commonly located on the anterior region of the hip joint. The results help establish motion capture as a potential method for quantitatively assessing the outcome in FAI surgical interventions.
Gait kinematic changes with aging have been used to assess the risk of developing knee OA with age.13 Specifically, the angle of knee flexion at heel strike (the beginning of stance phase) is typically near zero or in slight extension (Figure 5) in a younger (29±4 years) population. In contrast, a healthy older (59±9 years) population showed a higher knee flexion at heel strike that was similar to patients with mild knee OA.
Interestingly, patients with more severe knee OA had an even higher knee flexion angle at heel strike (Figure 5). Given the fact that aging is the highest risk factor for knee OA, these data (Figure 5) suggest an association between the angle of knee flexion at heel strike and the development of knee OA. As such, one could assume that increasing knee flexion at heel strike during gait is a marker of the risk for developing knee OA.
Interestingly, patients with more severe knee OA had an even higher knee flexion angle at heel strike (Figure 5). Given the fact that aging is the highest risk factor for knee OA, these data (Figure 5) suggest an association between the angle of knee flexion at heel strike and the development of knee OA. As such, one could assume that increasing knee flexion at heel strike during gait is a marker of the risk for developing knee OA.
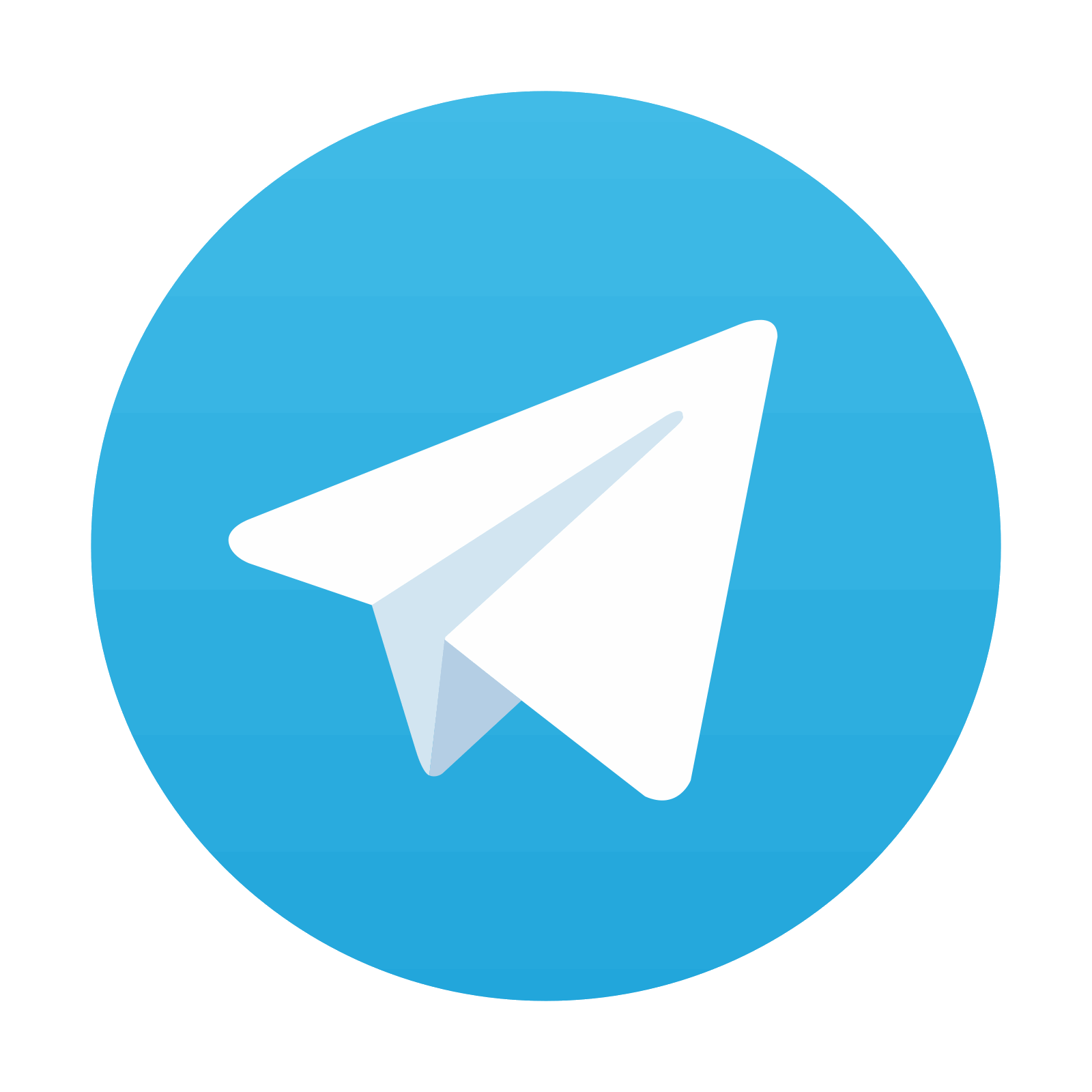
Stay updated, free articles. Join our Telegram channel
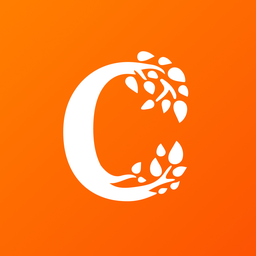
Full access? Get Clinical Tree
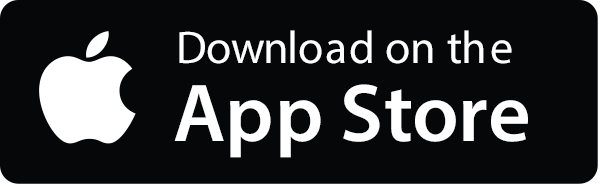
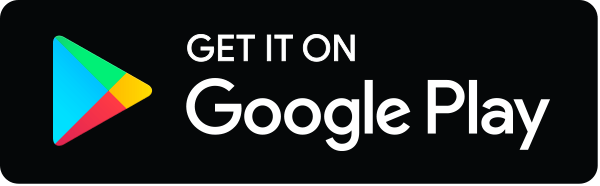