25 Future advances in the treatment of articular cartilage defects will be based on improved understanding of chondrocyte biology, early detection of cartilage injury, and functional tissue engineering. Chondrocyte resuscitation following mechanical injury may assist in preventing net proteoglycan loss leading to structural breakdown. This can reduce both the incidence and the size of chondral defects. Larger, symptomatic defects will need reconstruction with tissue engineering methods that yield biomechanically sound neocartilage. New imaging methods allowing for nondestructive assessment of cartilage microarchitecture and metabolic capacity will assist in early diagnosis of compromised articular cartilage. These same methods can be used for longitudinal assessment of articular cartilage treatment modalities. Advanced Magnetic Resonance Imaging Magnetic resonance imaging (MRI) has revolutionized medical imaging of the musculoskeletal system.1 Noninvasive and direct visualization of bone, marrow, and supporting soft tissue structures can be obtained with this technology, and the procedure is well tolerated by patients, especially after the development of open MRI systems. MRI offers a multiplanar tomographic viewing perspective, eliminating projectional distortion and magnification as well as the problem of superimposing of overlaying structures. Techniques describing articular cartilage with conventional MRI have been developed, and studies have shown that these techniques are both sensitive and specific for detecting articular cartilage defects in the knee.2 A study revealed that in patients with hip dysplasia conventional radiography showed no evidence of joint space narrowing; however, MRI detected articular cartilage defects in almost half of the examined patients.3 Furthermore, techniques for quantifying the volume of articular cartilage and for mapping its thickness have been developed and validated.4–6 However, the vast availability of MRI has affected the costs of health care in a significant manner, and studies have shown that a thorough clinical examination can be as successful in evaluating the status of articular cartilage as MRI. In one study, the diagnostic performance of clinical examination and MRI in the evaluation of intraarticular knee disorders was compared with intraoperative arthroscopic findings. The authors concluded that selective MRI does not provide enhanced diagnostic utility over clinical examination, particularly in children, and should be used cautiously.7 In recent years, more sensitive and specific MRI techniques have been developed for evaluating articular cartilage abnormalities. These techniques have focused on monitoring the concentration of glycosaminoglycans in articular cartilage, which are lost early in the course of osteoarthritis (OA) and, furthermore, are a major mechanical supporter of articular cartilage.8 Gadolinium-enhanced MRI techniques rely on the fact that gadolinium diethylenetriamine pentaacetic acid (Gd-DTPA2-) distributes into degraded areas at a higher concentration than into nondegraded areas. This technique was validated in cadaveric specimens.9 Gadolinium-enhanced MRI has also been evaluated in vivo, and the technique was proven feasible for imaging of the glycosaminoglycan concentration of articular cartilage.10 Studies have shown that T1 in the presence of Gd-DTPA2- differs by more than twofold when comparing regions of normal and osteoarthritic tissue. The contrast agent can be administered intraarticularly or intravenously, whereby intravenous delivery is about four times faster and Gd-DTPA2- is able to penetrate the articular cartilage both from the bone—cartilage as well as the synovial fluid-cartilage interfaces. Intravenous administration is associated with less patient discomfort. However, it involves a higher systemic dose and is more costly than intraarticular administration. Triple-quantum-filtered sodium MRI has been proposed as a method to discriminate between intracellular and extracellular sodium. However, a low signal-to-noise ratio has been a major obstacle to triple-quantum-filtered sodium MRI. In one study, the results of phantom experiments showed that a gradient-echo multiple-quantum-filtered 23Na imaging sequence produces the highest signal-to-noise ratio. The sensitivity of triple-quantum-filtered sodium MRI to ischemia was furthermore demonstrated in brains of live canines.11 This technique was also applied in vivo in healthy volunteers to demonstrate its feasibility in imaging of human articular cartilage. Triple-quantum-filtered sodium MRI images clearly demarcated patellar cartilage and demonstrated fluid signal suppressed by the triple quantum filter.12 FIGURE 25–1 Optical coherence tomography (OCT) of articular cartilage. OCT is a new imaging technique that can be used arthroscopically to obtain histology resolution cross-sectional images of articular cartilage instantaneously. (A) Arthroscopic view of OCT probe (arrow). (B) Cross-sectional OCT image of healthy appearing articular cartilage (See Color Plate 25–1B). Optical Coherence Tomography Optical coherence tomography (OCT) is an optical imaging technique that allows for nondestructive high-resolution cross-sectional imaging of articular cartilage.13 Therefore, OCT has the potential to be used for early diagnosis of cartilage damage. OCT was first introduced to image the eye.14 The technology can be described as similar to ultrasound except that the image generated is an echograph of infrared light instead of ultrasound. OCT has been applied to a wide variety of tissues to obtain high-resolution cross-sectional images comparable to low power histology and superior to both conventional MRI (150 μm) and ultrasound.15 When applied to assessment of tissue engineered cartilage repair, OCT imaging was found to be comparable to low-power histology (Fig. 25–1).16 Unlike histology in which the diseased tissue must be removed for processing and analysis, the OCT “biopsy” was obtained in situ and in near real time with no damage to the cartilage repair. The ability to obtain high-resolution nondestructive images of articular cartilage using OCT may result in earlier and more accurate diagnosis of articular cartilage injury. Biomechanical Probes Articular cartilage functions as a near frictionless, load-bearing tissue. Assessment of the compressive properties of the tissue may assist clinicians in determining the health of the articular cartilage. This information may also be useful in determining whether the repair tissue following a cartilage regenerative procedure is sufficiently strong to permit an athlete to return to sports. Indentation testing is a common method used to evaluate the compressive behavior of articular cartilage.17 Several handheld indentation probes have been developed to measure in situ compressive stiffness.18,19 One electromechanical probe (Artscan 1000, Artscan, Ltd, Helsinki, Finland) has been used to assess repair tissue stiffness in patients after autologous chondrocyte implantation.20 This device measures the resistance of the cartilage to a short deformation applied by an indenter. Although this type of indenter provides information on surface and subsurface stiffness, the device cannot measure or account for the important effects of cartilage thickness on the material properties of the tissue.21–23 Ultrasound devices enable measurement of cartilage thickness, and have some capacity to assess structure and composition.24–27 A system that was developed at the University of Pittsburgh was validated utilizing bovine knee specimens. The true sound speed in articular cartilage was measured using a miniature ultrasound contact transducer and correlated with the indentation stiffness. The authors found a significant decrease in indentation stiffness for proteoglycan-depleted specimens compared with normal articular cartilage.28 Chondrocyte injury and cartilage structural damage has been shown to occur following chemical, mechanical, and thermal injury. To evaluate the chondroprotective effects of interventions ranging from controlled passive motion to growth factors, antioxidants and heat treatment, appropriate models need to be developed at the cellular, tissue and organ levels. In relation to athletic injuries and arthroscopic surgery, the most relevant models involve impact injury and thermal stress. Mechanical Injury Models Early observations on decreased diffusion capacity of continuously compressed articular cartilage lead to the first impact injury models in articular cartilage research.29 The classic impact injury model was described by Thompson and Bassett30 in 1970. In this work, degeneration of articular cartilage was experimentally induced by compression immobilization and condylar resection. The survival of human articular cartilage was critically impaired by strains exceeding 20 to 25%.31,32 By applying subfracture loads to human patellofemoral joints, horizontal split fractures in subchondral bone, and at the interface of calcified cartilage and subchondral bone could be observed.33 The magnitude of cell damage was studied utilizing an impact injury model and is characterized by increased release of proteoglycans from the specimens subjected to stress.34 Excess mechanical stress has been shown to result in chondrocyte apoptosis, decreased proteoglycan synthesis, and matrix breakdown. Most current mechanical injury models focus on either static compression or cyclic loading. Although these models result in chondrocyte injury, it has been difficult to adequately model the effects of a single sudden impact similar to that of an anterior cruciate ligament (ACL) injury or subchondral fracture. Controlled Thermal Injury Thermal devices have gained widespread clinical use in the arthroscopic treatment of intraarticular pathology. There is great need for safe and effective arthroscopic methods to remove and stabilize damaged articular cartilage. The use of thermal energy to perform chondroplasty has sparked both interest and controversy. Histologically, radiofrequency energy (RFE) chondroplasty has been reported to deliver a smoother residual surface than mechanical shavers. Although RFE chondroplasty has been reported to deliver superior clinical results to shaver debridement in at least one study, the procedure has become increasingly controversial because of concerns over excessive collateral chondrocyte death from thermal injury. Quantifying the effects of thermal stress on articular cartilage is important both in evaluating the safety of intraarticular use of thermal devices and in the potential development of a well-defined chondrocyte injury model. After examining the effects of time and temperature on both osteoarthritic and normal human articular cartilage, it has been determined that chondrocytes possess the ability to recover from short-term exposures to high temperatures. Arthritic cartilage was found to be more sensitive to increased thermal exposure than nonarthritic cartilage. Additionally, articular cartilage was found to transiently increase proteoglycan synthesis following mild temperature elevation. FIGURE 25–2 Computer-controlled treatment stage (See Color Plate 25–2B). The use of a computer-controlled treatment stage allows for the production of discrete, reproducible levels of articular cartilage injury (Fig. 25–2). Further development of this thermal injury model will allow for controlled in vitro and in vivo experiments to evaluate the effects of different depths of chondrocyte injury on the structural, metabolic and functional properties of articular cartilage. Controlled thermal stress also provides a means to study ways to optimize survival of the stressed chondrocyte. Although chondrocytes make up less than 10% of articular cartilage, they are responsible for maintenance of the glycosaminoglycan-rich matrix. Under normal physiologic conditions, chondrocytes exhibit low metabolic activity, divide infrequently, and have a long life. The stressed chondrocyte increases metabolic activity, clones itself, and may undergo apoptosis. Optimizing survival and recovery of the stressed chondrocyte represents an active area of research with long-ranging potential toward acute treatment of cartilage injury and the prevention of osteoarthritis. Chondrocyte Resuscitation The concept of chondroprotection is of great importance in reducing cartilage damage after injury and in slowing, perhaps even preventing or reversing, the progression of degenerative arthritis. Recent studies from our laboratories demonstrate that the tolerance of articular cartilage for longer periods at higher temperatures may be improved in the presence of insulinlike growth factor-I (IGF-I) and an inhibitor of c-Jun N-terminal kinase (JNK) or stress activated protein kinase. The JNK pathway is considered important in stress-induced cell death through apoptosis. Both IGF-I and JNK inhibition improved metabolic recovery of human articular cartilage after thermal injury. In addition to having anabolic effects on articular cartilage, IGF-1 has been shown to have antiapoptotic effects.35 Apoptosis is an ordered process known as “programmed cell death” important to morphogenesis and tissue homeostasis.36 More recently, experimental evidence indicates that cells injured by environmental stress and cells in pathologic tissues such as osteoarthritic cartilage also undergo apoptosis.37,38 The JNK pathway is an important signal transduction mechanism for converting stress signals into apoptosis signaling in a variety of cell types.39,40 That improved recovery of cartilage metabolic activity after thermal stress was seen after the addition of two different antiapoptotic agents suggests that stress-induced apoptosis may be involved in chondrocyte necrosis following thermal injury. These studies demonstrate the great potential for the future use of substances such as JNK inhibitor and cartilage-friendly growth factors such as IGF-I to resuscitate damaged chondrocytes. Gene Therapy Growth factors are proteins that can be liberated by cells at the injury site (e.g., fibroblasts, endothelial cells, muscle cells, mesenchymal stem cells) and by the infiltrating reparatory or inflammatory cells (e.g., platelets, macrophages). They are capable of stimulating cells toward proliferation, migration, matrix synthesis, and differentiation.41–49 With the help of recombinant DNA technology, using the specific gene encoding the proteins, it is now possible to synthesize large quantities of the therapeutic proteins for the purpose of treatment. The direct application of human recombinant therapeutic proteins displays effects on the healing process; however, its application is limited by their short biologic half-life and by the need for repeated and high dosages. Therefore, the most difficult question to resolve prior to starting a gene therapy trial is what delivery method to choose. The goal of the delivery is to get the DNA that encodes for the specific protein into the target cell so that the cell will start expressing the growth factor. The most commonly used method is a viral insertion of the DNA into the target cell, which is called transduction.50–52 Alternatively, there are nonviral methods, such as “naked DNA” or liposomal vectors. The transferred gene is either integrated into the chromosomal DNA (e.g., retroviral transfection) or maintained separately in the cell as an episome (e.g., adenoviral transfection).53 For expression, the desired gene has to enter the pathways of transcription [copying the DNA into messenger RNA (mRNA)], translation (actual protein synthesis according to the mRNA template), and secretion of the therapeutic protein (e.g., growth factor). This process can be repeated so that the genetically manipulated cells serve as a reservoir for growth factors improving healing. Gene therapy was originally for the manipulation of germline cells for treating inherited genetic disorders, but this application has been limited due to considerable ethical concern. Conversely, the manipulation of somatic cells has been widely accepted. However, the recently reported cases of the development of leukemialike side effects of clinical trials for the treatment of children with severe combined immunodeficiency (SCID) have raised concerns about the risk-benefit ratio of gene therapy. Thus, research addressing safety issues is invaluable, especially in the field of orthopaedic sports medicine, where elective surgeries are performed on a relatively young population.54,55 Strategies for local gene therapy have been extensively investigated in the field of sports medicine. Vectors can be directly injected in the host tissue, or cells in culture can be genetically altered with a vector (ex vivo) and transplanted.56 Although the direct method is technically easier to achieve, the cell-based ex vivo approach bares less risk, because gene manipulation occurs outside the body of the host. Furthermore, the genetically engineered and transplanted cells supply the host with the desired gene and with cells responding and participating in the healing process. Decreased gene expression over time is common and its mechanisms are not fully understood. However, in certain tissues such as articular discs of the spine, gene expression could be observed in rabbits for more than 12 months.57 Currently research is focusing on regulating gene expression. Specifically, the induction of gene expression could help to control the gene expression and modulate implanted genes while turning them on and off. Certain promoter sequences that have been identified include a promoter that is activated only when animals have tetracycline in their diet and a promoter that is inducible by x-ray irradiation.58,59 At the experimental level, the feasibility of gene therapy was shown to the ligament insertion, meniscus, articular cartilage, and synovial tissue of the knee joint.60,61 Beyond this stage, experimental research in animal models has revealed that ligament insertions can be enhanced through gene therapy, that muscle scarring can be diminished in muscle laceration, that knee joint arthritis can be decreased, and that muscle-derived stem cells promote the healing of cartilage lesions. Cartilage has a low potential for healing. Injuries may lead to premature arthritis and a decrease in the quality of life, and it has enormous long-term health care costs. Growth factors such as bone morphogenetic protein—2 (BMP-2), basic fibroblast growth factor (bFGF), transforming growth factor-( (TGF-(), IGF-I, and cartilage-derived morphogenetic protein (CDMP) have both demonstrated in vitro and in vivo beneficial effects on cartilage healing.62,63 Muscle scaffolds in combination with myoblasts and IGF-I have been successfully used to induce healing in a rabbit articular cartilage injury model (Fig. 25–3).64 In addition to autologous chondrocytes, stem cells from peripheral tissues such as muscle or bone marrow represent potential sources for cell transplantation, which can be used in conjunction with gene therapy approaches. Several gene therapy and tissue engineering approaches to cartilage lesions are currently under investigation. However, the most efficient methodology for healing of these lesions has not yet been established. Meniscus tears can be treated with surgical techniques such as sutures, arrows, or staples when they occur in the vascularized peripheral third of the meniscus. Meniscal lesions in the avascular central part do not heal. Experimental studies have shown that healing in the central meniscus parts might be promoted by stimuli derived from a fibrin clot, synovial tissue, or growth factors (transforming growth factor-(, bFGF, epidermal-derived growth factor).65,66 The aims of gene therapy approaches are to deliver these growth factors directly or with the help of cells. This could also be managed in a preconditioning approach. FIGURE 25–3 Cartilage full-thickness defect (A), after 6 weeks of healing with myoblasts and insulin-like growth factor-I. Although gene therapy is not yet established as a clinical therapy, it has great potential for the treatment of musculoskeletal injuries in the future. Phase I of the first clinical trial in orthopedics was successfully completed for human joints.56,67 It is believed that further tissue engineering with muscle-derived stem cells and gene therapy will lead to the development of new treatment strategies for tissues with low healing capacities such as articular cartilage. New techniques that address the biologic base of healing might further improve the outcome and create indications for surgical interventions in tissues with low healing potential. However, a large number of basic science studies and preclinical trials have to be completed to reach the necessary efficiency and safety for orthopedic applications. Functional Tissue Engineering Autologous chondrocyte implantation is the first Food and Drug Administration (FDA)-approved cell-based treatment for articular cartilage defects. The two-stage procedure involves arthroscopic harvest of ~200 mg of cartilage from the patient’s knee. The chondrocytes are isolated from the biopsy and expanded ex vivo into several million cells, which are then reimplanted into the defect under a piece of periosteum at second-stage arthrotomy. Future improvements to the reimplantation of autologous chondrocytes include substituting both natural and synthetic membranes for the periosteum. Membranes under consideration include collagen membranes, biodegradable membranes, and porcine intestine submucosal tissue. Another strategy is to seed the autologous chondrocytes into collagen gels, hydrogels, hyaluronic acid, biodegradable scaffolds, or injectable pastes and glues.68–75 Coaxing stem cells into chondrogenic repair cells represent another promising line of research for the repair of chondral defects. Stem cells are cells that can turn into different tissue types, such as bone, cartilage, muscle, or tendon. For example, fetal umbilical cord cells are stem cells that display the ability to differentiate in various tissue types. Unfortunately, they are not available in adults. In contrast, bone marrow—derived stem cells and muscle-derived stem cells persist throughout life, are available in abundance, and easily accessible through a biopsy. A special stem cell population derived from muscle tissue was identified, and tissue-engineering approaches are currently under development, which means biologic substitutes for repair, reconstruction, regeneration, or replacement of musculoskeletal tissues with muscle-derived stem cells.76 Functional tissue engineering is a novel approach to enhance tissue regeneration and provides the possibility of producing tissue that is biomechanically, biochemically, and histomorphologically similar to the normal.77,78 The basic concept of tissue engineering is based on the manipulation of cellular and biochemical mediators to affect protein synthesis and to improve tissue formation and remodeling. Ultimately, the process is expected to lead to a restoration of mechanical properties.79 Examples of the available approaches are the use of growth factors, gene transfer technology to deliver genetic material, stem cell therapy, and the use of scaffolding as well as external mechanical factors. Each of these approaches, or their combinations, offers the opportunity to enhance the healing process. Advances in regenerative medicine, cell biology, genomics, and biomaterials hold promise for development of effective treatment techniques for articular cartilage injuries. Although many partial solutions will likely make their way into clinical practice in the near future, treating cartilage-injured patients and athletes with arthritis will most likely remain a clinical challenge. It must be remembered that articular cartilage is an enigmatic tissue and that methods to repair articular cartilage have eluded scientists and physicians at least since Hippocrates’s time. The future, however, belongs to those with vision. We foresee cartilage restoration through comprehensive assessment, chondrocyte resuscitation, and functional tissue engineering. REFERENCES 1. Damadian R. Tumor detection by nuclear magnetic resonance. Biophys J 1971;11:739–760 2. Recht M, Pirraino D, Paletta G, Schils J, Belhobek G. Accuracy of fat-suppressed three-dimensional spoiled gradient-echo FLASH MR imaging in the detection of patellofemoral articular cartilage abnormalities. Radiology 1996;198:209–212 3. Nishii T, Sugano N, Tanako H, Nakarishi K, Ohzono K, Yoshikawa H. Articular cartilage abnormalities in dysplastic hips without joint space narrowing. Clin Orthop Relat Res 2001;383:183–190 4. Peterfy C, van Dijke CF, Janzen DL, et al. Quantification of articular cartilage in the knee with pulsed saturation transfer subtraction and fat-suppressed MR imaging: optimization and validation. Radiology 1994;192:485–491 5. Eckstein F, Winzheimer M, Hohe J, Englmeier K, Reiser M. Interindividual variability and correlation among morphological parameters of knee joint cartilage plates: analysis with three-dimensional MR imaging. Osteoarthritis Cartilage 2001;9:101–111 6. Cicuttini F, Forbes A, Asbeutah A, Morris K, Stuckey S. Comparison and reproducibility of fast and conventional spoiled gradientecho magnetic resonance sequences in the determination of knee cartilage volume. J Orthop Res 2000;18:580–584 7. Kocher M, DiCanzio J, Zurakowski D, Micheli L. Diagnostic performance of clinical examination and selective magnetic resonance imaging in the evaluation of intraarticular knee disorders in children and adolescents. Am J Sports Med 2001;29: 292–296 8. Frank EH, Grodzinsky AJ. Cartilage electromechanics—I. Electro-kinetic transduction and the effects of electrolyte pH and ionic strength. J Biomech 1987;20:615–627 9. Bashir A, Gray M, Burstein D. Gd(DTPA)2– as a measure of cartilage degradation. Magn Reson Med 1996;36:665–673 10. Bashir A, Gray M, Boutin R, Burstein D. Glycosaminoglycan in articular cartilage: in vivo assessment with delayed Gd(DTPA)2-enhanced MR imaging. Radiology 1997;205:551–558 11. Kalyanapuram R, Seshan V, Bansal N. Three-dimensional triple-quantum-filtered 23Na imaging of the dog head in vivo. J Magn Reson Imaging 1998;8:1182–1189 12. Borthakur A, Hancu I, Boada FE, Shen GX, Shapiro EM, Reddy R. In vivo triple quantum filtered twisted projection sodium MRI of human articular cartilage. J Magn Reson 1999; 141: 286–290 13. Herrmann JM, Pitris C, Bouma BE, et al. High resolution imaging of normal and osteoarthritic cartilage with optical coherence tomography. J Rheumatol 1999;26:627–635 14. Huang D, et al. Optical coherence tomography. Science 1991; 254:1178–1181 15. Pan Y, Lavelle JP, Bastacky SI, et al. Detection of tumorigenesis in rat bladders with optical coherence tomography. Med Phys 2001;28:2432–2440 16. Han C, Chu CR, Adachi N, et al. Analysis of rabbit articular cartilage repair after chondrocyte implantation using optical coherence tomography. Osteoarthritis Cartilage 2003;11:111–121 17. Athanasiou KA, Niederauer GG, Schenck RC Jr. Biomechanical topography of human ankle cartilage. Effects of excimer laser on healing of articular cartilage in rabbits. Ann Biomed Eng 1995;23:697–704 18. Appleyard RC, Burkhardt D, Ghosh P, et al. Topographical analysis of the structural, biochemical and dynamic biomechanical properties of cartilage in an ovine model of osteoarthritis. Osteoarthritis Cartilage 2003;11:65–77 19. Toyras J, Lyyra-Laitinen T, Niinimaki M, et al. Estimation of the Young’s modulus of articular cartilage using an arthroscopic indentation instrument and ultrasonic measurement of tissue thickness. J Biomech 2001;34:251–256 20. Peterson L, Lindahl A, Brittberg M, Nilsson A. Durability of autologous chondrocyte Transplantation of the knee. In: 67th annual meeting of the American Academy of Orthopaedic Surgeons, Orlando, FL, 2000 21. Jurvelin JS, Vaatainen U, Helminen HJ, Wong M. Volumetric changes of articular cartilage during stress relaxation in unconfined compression. Scand J Med Sci Sports 2000;10:186–198 22. Mow VC, Gibbs MC, Lai WM, Zhu WB, Athanasiou KA. Biphasic indentation of articular cartilage—II. A numerical algorithm and an experimental study. Material properties of the normal medial bovine meniscus. J Biomech 1989;22:853–861 23. Chen FS, Frenkel SR, Di Cesare PE. Chondrocyte transplantation and experimental treatment options for articular cartilage defects. Am J Orthop 1997;26:396–406 24. Cherin E, et al. Ultrasonic propagation properties of articular cartilage at 100 MHz. J Bone Miner Res 1997;12:1378–1386 25. Cherin E, Saied A, Pellaumail B, et al. Assessment of rat articular cartilage maturation using 50-MHz quantitative ultrasonography. Osteoarthritis Cartilage 2001;9:178–186 26. Seedhom BB, Yao JQ. Ultrasonic measurement of the thickness of human articular cartilage in situ. Clin Biomech (Bristol, Avon) 1999;14:166–176 27. Toyras J, et al. T2 relaxation reveals spatial collagen architecture in articular cartilage: a comparative quantitative MRI and polarized light microscopic study. Ultrasound Med Biol 2002;28: 519–525 28. Youn I, Fu F, Suh J. Determination of the indentation stiffness of articular cartilage using a high-frequency ultrasonic indentation technique. Pittsburgh Orthop J 1999;10:159–160 29. Salter RB, Field P. The effects of continous compression on living articular cartilage. An experimental investigation. J Bone Joint Surg 1960;42-A:31–90 30. Thompson RC Jr, Bassett CA. Histological observations on experimentally induced degeneration of articular cartilage. J Bone Joint Surg Am 1970;52:435–443 31. Repo RU, Finlay JB. Survival of articular cartilage after controlled impact. J Bone Joint Surg Am 1977;59:1068–1076 32. Radin EL, Paul IL, Lowy M. A comparison of the dynamic force transmitting properties of subchondral bone and articular cartilage. J Bone Joint Surg Am 1970;52:444–456 33. Atkinson PJ, Ewers BJ, Haut RC. Blunt injuries to the patellofemoral joint resulting from transarticular loading are influenced by impactor energy and mass. J Biomech Eng 2001;123: 293–295 34. Quinn TM, Allen RG, Schalet BJ, Perumbuli P, Hunziker EB. Matrix and cell injury due to sub-impact loading of adult bovine articular cartilage explants: effects of strain rate and peak stress. J Orthop Res 2001;19:242–249 35. Parrizas M, Saltiel AR, LeRoith D. Insulin-like growth factor 1 inhibits apoptosis using the phosphatidylinositol 3’-kinase and mitogen-activated protein kinase pathways. J Biol Chem 1997;272: 154–161 36. Majno G, Joris I. Apoptosis, oncosis, and necrosis. An overview of cell death. Am J Pathol 1995;146:3–15 37. Lotz M, Takahashi K. Effect of hyaluronan on chondrocyte apoptosis and nitric oxide production in experimentally induced osteoarthritis. Arthritis Rheum 2001;44:1644–1653 38. D’Lima DD, Hashimoto S, Chen PC, Lotz MK, Colwell CW Jr. In vitro and in vivo models of cartilage injury. Cartilage injury induces chondrocyte apoptosis. J Bone Joint Surg Am 2001;83-A(suppl 2):22–24 39. Davis RJ. Signal transduction by the JNK group of MAP kinases. [comment] Cell 2000;103:239–252 40. Zanke BW, Boudreau K< Pubie E, et al. The stress-activated protein kinase pathway mediates cell death following injury induced by cis-platinum, UV irradiation or heat. Curr Biol 1996;6:606–613 41. Yablonka-Reuveni Z, Balestreri TM, Bowen-Pope DF. Regulation of proliferation and differentiation of myoblasts derived from adult mouse skeletal muscle by specific isoforms of PDGF. J Cell Biol 1990;111:1623–1629 42. Menetrey J, Kasemkijwattana C, Day CS, et al. Growth factors improve muscle healing in vivo. J Bone Joint Surg Br 2000;82:131–137 43. Schofield JN, Wolpert L. Effect of TGF-beta 1, TGF-beta 2, and bFGF on chick cartilage and muscle cell differentiation. Exp Cell Res 1990;191:144–148 44. Shuler FD, Georgescu H, Niyibizi C, et al. Increased matrix synthesis following adenoviral transfer of a transforming growth factor beta-1 gene into articular chondrocytes. J Orthop Res 2000;18:585–592 45. Sellers RS, Zhang R, Glasson SS, et al. Repair of articular cartilage defects one year after treatment with recombinant human bone morphogenetic protein-2 (rhBMP-2). J Bone Joint Surg Am 2000;82:151–160 46. Hunziker EB, Rosenberg LC. Repair of partial-thickness defects in articular cartilage: cell recruitment from the synovial membrane. J Bone Joint Surg Am 1996;78:721–733 47. Isgaard J, Nilsson A, Lindahl A, Jansson JO, Isaksson OG. Effects of local administration of GH and IGF-1 on longitudinal bone growth in rats. Am J Physiol 1986;250:E367–E372 48. Kasemkijwattana C, Menetry J, Bosch P, et al. Use of growth factors to improve muscle healing after strain injury. Clin Orthop Relat Res 2000;370:272–285 49. Lieberman JR, Le LQ. WU L, et al. Regional gene therapy with a BMP-2-producing murine stromal cell line induces heterotopic and orthotopic bone formation in rodents. J Orthop Res 1998;16:330–339 50. Hannallah D, Peterson B, Lieberman JR, Fu F, Huard J. Gene therapy in orthopaedic surgery. J Bone Joint Surg Am 2002;84:1046–1061 51. Robbins PD, Ghivizzani SC. Viral vectors for gene therapy. Pharmacol Ther 1998;80:35–47 52. Robbins PD, et al. Retroviral vectors for use in human gene therapy for cancer, Gaucher disease, and arthritis. Ann N Y Acad Sci 1994;716:72–88; discussion 88–89 53. Musgrave DS, Bosch P, Ghivizzani S, Robbins PD, Evans CH, Huard J. Adenovirus-mediated direct gene therapy with bone morphogenetic protein-2 produces bone. Bone 1999;24:541–547 54. Marshall E. Gene therapy. Second child in French trial is found to have leukemia. Science 2003;299:320 55. Hacein-Bey-Abina S, von Kall C, Schmidt M, et al. A serious adverse event after successful gene therapy for X-linked severe combined immunodeficiency. N Engl J Med 2003;348:255–256 56. Evans C, Robbins P. Possible orthopaedic application of gene therapy. J Bone Joint Surg Am 1995;77:1103–1114 57. Kang R, Ghivizzani S, Muzzonigro TS, Herndon JH, Robbins PD, Evans CH. The Marshall R. Urist Young Investigator Award. Orthopaedic applications of gene therapy. From concept to clinic. Clin Orthop 2000;375:324–337 58. Gossen M, Bujard H. Tight control of gene expression in mammalian cells by tetracycline-responsive promoters. Proc Natl Acad Sci U S A 1992;89:5547–5551 59. Worthington J, Robson T, Murray M, O’Rourke M, Keilty G, Hirst DG. Modification of vascular tone using iNOS under the control of a radiation-inducible promoter. Gene Ther 2000;7: 1126–1131 60. Martinek V, Latterman C, Usas A, et al. Enhancement of tendonbone integration of anterior cruciate ligament grafts with bone morphogenetic protein-2 gene transfer: a histological and biomechanical study. J Bone Joint Surg Am 2002;84-A:1123–1131 61. Adachi N, Sato K, Usas A, et al. Muscle derived, cell based ex vivo gene therapy for treatment of full thickness articular cartilage defects. J Rheumatol 2002;29:1920–1930 62. Morales I, Roberts A. Transforming growth factor-β regulates the metabolism of proteoglycans in bovine cartilage organ cultures. J Biol Chem 1988;263:12828–12831 63. Tyler J. Insulin-like growth factor-1 can decrease degradation and promote synthesis in cartilage exposed to cytokines. Biochem J 1989;260:543–548 64. Lee CW, et al. Myoblast mediated gene therapy with muscle as a biological scaffold for the repair of full-thickness defects of articular cartilage. In: 25th annual meeting of the Orthopaedic Research Society, vol. 25. Orlando, FL, 2000:1068 65. Martinek V, Usas A, Pelinkovic D, Robbins P, Fu FH, Huard J. Genetic engineering of meniscal allografts. Tissue Eng 2002;8: 107–117 66. van Tienen TG, Heijkants RG, Buma P, de Groot JH, Pennings AJ, Veth RP. Tissue ingrowth and degradation of two biodegradable porous polymers with different porosities and pore sizes. Biomaterials 2002;23:1731–1738 67. Evans CH, Ghivizzani SC, Herndon JH, et al. Clinical trials in the gene therapy of arthritis. Clin Orthop 2000;379:S300–S307 68. Freed LE, Marquis JC, Nohria A, mmanual J, Mikos AG, Langer R. Neocartilage formation in vitro and in vivo using cells cultured on synthetic biodegradable polymers.J Biomed Mater Res 1993;27: 11–23 69. Badylak SF, Park K, Peppas N, McCabe G, Yoder M. Marrow-derived cells populate scaffolds composed of xenogeneic extracellular matrix. Exp Hematol 2001;29:1310–1318 70. Caplan AI. Tissue engineering designs for the future: new logics, old molecules. Tissue Eng 2000;6:1–7 71. Marcacci M, Zaffagnini S, Kon E, Visani A, Iacono F, Loreti I. Arthroscopic autologous chondrocyte transplantation: technical note. Knee Surg Sports Traumatol Arthrosc 2002;10:154–159 72. Knudson CB, Knudson W. Hyaluronan-binding proteins in development, tissue homeostasis, and disease. FASEB J 1993;7: 1233–1241 73. Martin I, Shastri VP, Padera RF, et al. Selective differentiation of mammalian bone marrow stromal cells cultured on three-dimensional polymer foams. J Biomed Mater Res 2001;55:229–235 74. Kawamura S, Wakitani S, Kimura T, et al. Articular cartilage repair. Rabbit experiments with a collagen gel-biomatrix and chondrocytes cultured in it. Acta Orthop Scand 1998;69:56–62 75. Wakitani S, Goto T, Young RG, Mansour JM, Goldberg VM, Caplan AI. Repair of large full-thickness articular cartilage defects with allograft articular chondrocytes embedded in a collagen gel. Tissue Eng 1998;4:429–444 76. Huard J, Ascadi G, Jani A, et al. Gene transfer into skeletal muscles by isogenic myoblasts. Human Gene Ther 1994;5:949–958 77. van der Meulen MC, Huiskes R. Why mechanobiology? A survey article. J Biomech 2002;35:401–414 78. Guilak F. Functional tissue engineering: the role of biomechanics in reparative medicine. Ann N Y Acad Sci 2002;961:193–195 79. Woo SL, Hildebrand K, Watanabe N, Penwick JA, Papageorgiou CD. Tissue engineering of ligament and tendon healing. Clin Orthop 1999;367:S312–S323 80. Martinek V, Fu FH, Huard J. Gene Therapy and Tissue Engineering in Sports Medicine. Phys Sportsmed 2000;28:34–51
Future Directions in the Treatment of Cartilage Injury in the Athlete
New Assessment Techniques
Improved Injury Models
Future Treatments
Conclusion
< div class='tao-gold-member'>
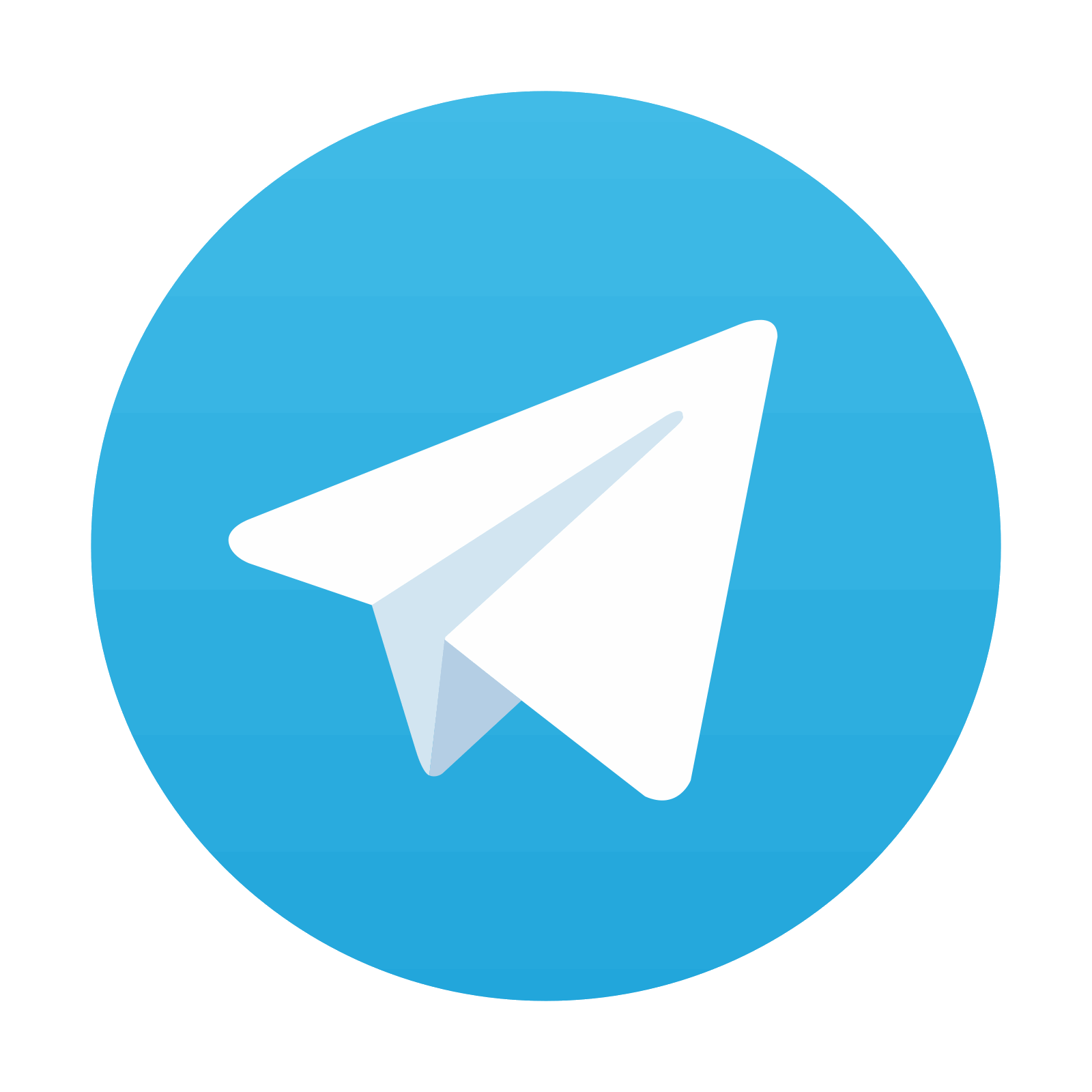