Fig. 10.1
Cartoon representation of ventral (left), lateral (top right), and medial (bottom right) of the human brain with Petrides and Pandya (1994) cytoarchitectonic delineation [adapted from Stuss et al. (2002)] and the evolutionary developmental trends with coding for the archicortical (white) and paleocortical (gray) cortical moieties and the cortical types within each trend [adapted after Giaccio (2006)]. Geometrical designs represent the percent of neuroimaging studies reporting activation differences between PTSD and controls on emotional/traumatic tasks and stimuli. Black geometric designs represent left-sided differences and white designs represent right-sided differences. The inferior parietal lobule is marked with an * because it is considered a transitional cortex belonging to both developmental trends (Pandya et al., 1988)

Fig. 10.2
Cartoon representation of ventral (left), lateral (top right), and medial (bottom right). Color and geometrical coding are identical to Fig. 10.1 except that geometrical designs represent the percent of neuroimaging studies reporting activation differences between PTSD and controls on neutral tasks and stimuli. Black geometric designs represent left-sided differences and white designs represent right-sided differences. The inferior parietal lobule is marked with * because it is considered a transitional cortex belonging to both developmental trends (Pandya et al., 1988)
The archicortical trend originates from the hippocampus. It then passes along the medial aspect of the temporal lobes with periallocortical, and proisocortical, parahippocampal cortices. This then gives rise to structures in the mediodorsal aspects of the parietal and frontal cortex, including proisocortical posterior cingulate cortex (Brodmann areas 31 and 23 posteriorly and 24, 25, and 32 anteriorly). Finally, this trend forms isocortical areas in the dorsomedial and dorsolateral prefrontal cortex (BA 9, 10, 46, and 8) (Pandya et al., 1988).
The paleocortical trend is differentiated from its limbic piriform/amygdala core, and journeys posteriorly along the entire temporal cortex from the proisocortical temporal pole, and progressively differentiates, as it extends caudally all the way to the caudalmost section of the temporal gyri. Anteriorly, the paleocortical trend differentiates into the proisocortical regions of the orbital and insular cortices, and the isocortical regions of the ventrolateral and lateral orbitofrontal cortex including Brodmann areas 10, 12, 46, 14, 8, and 11 (Pandya et al., 1988).
The functional significance of these two developmental trends for PTSD is discussed extensively below. Broadly, the two systems may be considered as serving distinctive roles in shaping and controlling behavior. The dorsal archicortical system, arising from the hippocampal area, is particularly suited for processing of spatial–temporal and contextual elements in the environment. The hippocampus best epitomizes this role as it specializes in binding items together to create novel arbitrary associations, and in spatial navigation. The ventral paleocortical system, arising from the olfactory cortex and amygdala, mediates the processing of object identity and the allocation of incentive significance to stimuli and supports motivational components of behavior. The centrality of neural systems that mediate motivational and reinforcement aspects of behavior, such as the amygdala and orbitofrontal cortex, exemplifies this role. Thus, evolutionary brain organization provides the basic architecture that supports independent processing of objects, and of contextual and spatial information, while maintaining synchronous representations of these processes. This structural design is reflected in two separate cortical systems that are also highly interconnected, allowing for efficient coordination. Both archicortical and paleocortical evolutionary trends have access to perimotor cortical structures at multiple levels, allowing them to control behavior through frontal executive neural networks. For example, the dorsal prefrontal cortex, which is part of the archicortical dorsal route, has extensive connections with supplementary motor/premotor areas and may guide complex behaviors that require temporal structuring and cognitive planning. The ventrolateral motor system, with its paleocortical limbic base, may mediate an affective influence over behavior through extensive connections with the amygdala and insular and temporal pole cortices. Moreover, the intensive connectivity of this ventral system with the insula may be important for linking visceroautonomic associations to perceptual events and to the organization of action plans.
The dual cytoarchitectonic trends framework can be instructive for understanding the functional neuroanatomy of psychiatric disorders. Most advanced neuropsychiatric models emphasize aberrations at the level of large network interactions, and imbalances among remote brain regions, rather than deficits associated with particular brain regions. The phylogenetic and ontogenetic neurodevelopmental model presented here has recently begun to filter into the psychiatric literature, primarily as a model for understanding Schizophrenia, but also as a more general model of understanding psychiatric disorders (Antonova, Sharma, Morris, & Kumari, 2004; Christensen & Bilder, 2000; Giaccio, 2006).
Next, I briefly review current findings from structural and functional neuroimaging involving patients suffering from PTSD. I will then present these findings within the conceptual framework of the dual developmental trends. It is important to note that despite theoretical advancement, many functional and structural neuroimaging studies do not possess the methodological complexity that could directly support system-level theories. Therefore, analyses are still conducted in a voxel-wise manner, or focusing on regions of interest. Thus I will separately discuss findings from functional and effective connectivity, studies that may hold particular promise for our understanding of the neural basis of PTSD.
Structural Findings in PTSD
The first report of PTSD-related neural structural abnormalities revealed reduced MRI-derived left hippocampal volume in Vietnam veterans with PTSD, compared with non-PTSD controls (Bremner et al., 1995). These findings had been interpreted as reflecting the vulnerability of this structure to the effects of stress hormones, within the framework of Gluccocorticoid Cascade Hypothesis (Sapolsky, Krey, & McEwen, 1986). Thus, it has been suggested that smaller hippocampal volumes reflect an acquired trait. The rationale behind this claim is that PTSD involves a prolonged state of stress, which leads to loss of hippocampal neurons. As described above, the hippocampus is the core of the archicortical (dorsomedial) developmental trend that mediates the processing of spatiotemporal aspects of behavior. It has been described as ”projectional,” in that it mediates action derived from internal probabilistic models of the future, based on previous experiences.
The pioneering report by Bremner et al. (1995) has prompted a surge of studies investigating structural abnormalities in PTSD, with particular focus on the hippocampus. Many of these studies have reported either left, right, or bilateral hippocampal volume reduction ranging from approximately 5 % to an incredible 26 % (Bonne et al., 2008; Bossini et al., 2008; Bremner et al., 1997, 2003; Emdad et al., 2006; Felmingham et al., 2009; Gurvits et al., 1996; Hedges et al., 2003; Lindauer et al., 2004; Shin et al., 2004; Stein, Koverola, Hanna, Torchia, & McClarty, 1997; Vermetten, Vythilingam, Southwick, Charney, & Bremner, 2003; Villarreal et al., 2002; Vythilingam et al., 2005; Wang et al., 2010; Woon & Hedges, 2008). For comparison, a volume reduction of 40 % is thought to reflect a nearly complete loss of hippocampal neurons in acquired amnesia in adults (Gold & Squire, 2005). Or, if we consider hippocampal volume reduction to be a vulnerability factor (see below) developmental amnesia resulting from anoxia-induced hippocampal atrophy involves volume loss of 20–30 % (Isaacs et al., 2003). Given that amnesia (acquired or developmental) involves a severe inability to acquire new declarative memories, one might wonder why in PTSD neuropsychological deficits in the realm of memory are only found in some studies, and why even these effects are rather modest.
Volumetric studies of hippocampal structural changes in PTSD have often reported significantly reduced volumes. However, this is by no means a universal finding. There are several reports that found no hippocampal volume difference (Bonne et al., 2001; Carrion et al., 2001; De Bellis, Hall, Boring, Frustaci, & Moritz, 2001; De Bellis et al., 2002; Golier et al., 2005; Jatzko et al., 2006). In addition, we consider the possibility that additional unpublished null result studies also exist. That said, recent meta-analyses further support the verity of reduced hippocampal volume in PTSD (Karl et al., 2006; Woon & Hedges, 2008). The inconsistencies across studies with regard to the existence, laterality, and the extent of hippocampal structural abnormalities, suggest that mediating or moderating factors must be taken into account. For example, smaller hippocampal volumes may be related to comorbid conditions such as depression or alcohol abuse, which are known to independently affect hippocampal integrity (Hedges & Woon, 2010; Videbech & Ravnkilde, 2004). In addition, hippocampal atrophy may be characteristic of only a subgroup of PTSD patients with particularly chronic and severe conditions. This latter possibility receives some support from a study of hippocampal volume in monozygotic twins discordant for PTSD diagnosis (Gilbertson et al., 2002). In that study, twins who had been to Vietnam and developed PTSD were found to have significantly smaller hippocampal volumes, but only when 5 of the 17 PTSD patients with the lowest CAPS score were removed from the analysis. Thus, even in this very chronic sample, significant hippocampal atrophy as measured by MRI could only be verified in the most affected individuals.
Gilbertson et al. landmark study was designed to address the etiology of reduced hippocampal volumes in PTSD. One possibility mentioned above is that smaller hippocampal volumes are an acquired trait mediated by elevated levels of glucocorticoids, either during trauma or later during the posttraumatic response. Another possibility is that smaller hippocampus is a vulnerability factor, and may be part of what determines the development of the disorder. Indeed why some individuals develop PTSD following traumatic experiences while others do not remains unresolved. Gilbertson and colleagues’ findings strongly support the latter possibility in that there were no differences between hippocampal volumes of PTSD patients and their non-traumatized twins, suggesting no disease-related atrophic processes. Moreover, there was a significant correlation between the hippocampal volumes of the unaffected siblings and the PTSD symptom severity of the affected twins. These findings strongly suggest smaller hippocampal volumes constitute a vulnerability factor, and point to the possibility that premorbid archicortical abnormalities lead to susceptibility to develop PTSD as a result of exposure to traumatic stress.
Structural abnormalities of the hippocampus in PTSD were the first to be reported and have received the most attention by far. However, more recent volumetric studies have also reported other morphometric differences associated with PTSD. These reports became more abundant with the advent of automatic methods for measuring brain volumes and atrophy. The primary gray matter regions reported in these studies as having reduced volumes in PTSD are the amygdala and regions within the anterior cingulate and the insula. There are also reports originating from pediatric PTSD investigations of white matter volume reductions primarily in the corpus callosum (De Bellis & Keshavan, 2003; De Bellis et al., 2002; Jackowski et al., 2008; Kitayama et al., 2007; Villarreal et al., 2004).
The amygdalae have been a target for structural investigations of PTSD from the outset, because of its role in emotional dysregulation in general, and particularly in fear conditioning. Most neurobiological models of PTSD have emphasized the role of the amygdala in the disorder’s pathophysiology and both functional and structural studies have attempted to uncover PTSD-related abnormalities in this structure. The evidence for amygdalar structural alterations associated with PTSD are quite weak (Bonne et al., 2001; Bremner et al., 1997; Carrion et al., 2001; Cohen et al., 2006; De Bellis et al., 2001; Fennema-Notestine, Stein, Kennedy, Archibald, & Jernigan, 2002; Gurvits et al., 1996; Hara et al., 2008; Karl et al., 2006; Lindauer et al., 2004; Matsuoka, Yamawaki, Inagaki, Akechi, & Uchitomi, 2003; Rauch et al., 2003; Rogers et al., 2009; Wignall et al., 2004; Woodward et al., 2006; Woon & Hedges, 2008, 2009) possibly because this structure is more difficult to reliably measure than the hippocampus. Accordingly, two recent meta-analyses reached somewhat different conclusions. One found that there is overall evidence for smaller left amygdala volumes in adults with PTSD compared with controls, but not in pediatric PTSD (Karl et al., 2006). The other study, on the other hand, found no significant volumetric differences in either population (Woon & Hedges, 2008), emphasizing the great inconsistencies and variability across studies.
The anterior cingulate cortex (ACC) has also been a target of structural investigation in PTSD. Its established role in extinction learning (Milad et al., 2006; Quirk & Beer, 2006) has led to several theories suggesting failed inhibition of amygdala activity is a central contributor to the pathophysiology of the disorder (Bremner, 2006b; Liberzon & Sripada, 2008; Rauch et al., 2006; Shin & Handwerger, 2009). Several studies have pointed to abnormal structural characteristics associated with PTSD in the anterior cingulate cortex (ACC). Patients with PTSD have been shown to have reduced ACC gray matter volumes compared with controls (Abe et al., 2006; Corbo, Clement, Armony, Pruessner, & Brunet, 2005; Kitayama, Quinn, & Bremner, 2006; Woodward et al., 2006; Yamasue et al., 2003) as well as white matter abnormalities of the cingulum bundle (Abe et al., 2006). These reduced volumes may be particularly characteristic of the rostral (~Brodmann area 32) and sub-callosal (~Brodmann area 25) portions of the ACC, rather than the dorsal ACC (Rauch et al., 2003). Rostral ACC volumes derived using VBM have also been found to predict response to cognitive-behavioral interventions such that larger volumes were associated with greater symptom reduction (Bryant et al., 2008). Finally a study of pregenual ACC volumes in monozygotic twins discordant for PTSD suggested reduced volumes may reflect an acquired trait of PTSD rather than a vulnerability factor (Kasai et al., 2008), in contrast with the findings of hippocampal volumes cited above.
One last cortical region that has been implicated in structural studies of PTSD is the insula. Reduced insular gray matter has been reported by several studies (Chen, Li, Xu, & Liu, 2009; Chen et al., 2006; Corbo et al., 2005; Kasai et al., 2008). However despite the proliferation of the use of whole-brain voxel-based morphometry in anatomical studies of PTSD, the evidence for insular structural deformities in PTSD appears to be very limited and awaits further research.
To conclude, more than 15 years of structural imaging in PTSD appear to indicate that the hippocampi, and less consistently the ACC, show abnormal morphology in the disorder. By contrast, abnormal structural findings in the amygdala and insular cortex have been inconsistently reported. Thus, of the two primordial core structures within the limbic ring, there seems to be better evidence for a structural abnormality associated with the dorsal-archicortical (hippocampus) than the ventral-paleocortical (amygdala) stream. Further down the archicortical stream, isocortical regions that belong to that system (i.e., the anterior ACC) also seem to be affected. Moreover, the structural deficit affecting the limbic core of the archicortical system appears to constitute a risk factor for developing the disorder, whereas further downstream, gray matter reduction in the ACC may represent an acquired sign of PTSD consistent with stress-induced loss.
Functional Imaging Findings
Current models of the neural basis of PTSD have greatly relied on evidence from human functional neuroimaging explorations of possible PTSD-related alterations in brain function. Functional neuroimaging studies include either studies of brain function at rest or studies of brain function during performance of tasks. The most prevalent task used in PTSD research is symptom provocation in response to processing of either individualized or generic trauma-related stimuli. However, many studies have also investigated possible neural alterations in processing and memory of generic emotional stimuli, as well as processing and memory of neutral stimuli. Several detailed reviews of these neuroimaging studies have been published in recent years (Bremner, 2006a, 2007; Cannistraro & Rauch, 2003; Deckersbach, Dougherty, & Rauch, 2006; Francati, Vermetten, & Bremner, 2007; Lanius, Bluhm, Lanius, & Pain, 2006; Liberzon & Sripada, 2008; Shin et al., 2006). Among others, these reviews have pointed to important heterogeneities in findings associated with factors such as subject characteristics, type of stimulus, type of imaging modality, and so forth. An extensive review is beyond the scope of the present chapter, but I attempt to sketch a fundamental picture of the functional neural systems that appear to be abnormally involved in the disorder. We (Shalev et al., 2011) have recently reviewed over 30 neuroimaging studies that have used emotional stimuli (Table 10.1; Fig. 10.1), and 15 studies that have used neutral stimuli and tasks (Table 10.2, Fig. 10.2). Frequencies of regions that are consistently reported as abnormally activated in PTSD compared with controls (regardless of whether this involves hypo- or hyperactivation) were plotted on brain cartoons that depict the two developmental trends. Although very general, this representation allows an overall appreciation of the relationship between functional disorders and cytoarchitectonic principles of brain structure.
Contrasts | Imaging modality | Study |
---|---|---|
Combat-related slides and sounds vs. neutral slides and sounds. | PET | Bremner et al. (1999) |
Script-driven imagery of autobiographical events: traumatic vs. neutral | PET | Bremner et al. (1999b) |
Retrieval of deeply encoded emotional words vs. neutral deeply encoded words | PET | Bremner et al. (2003b) |
Emotional stroop vs. neutral stroop | PET | Bremner et al. (2004) |
Script-driven imagery of autobiographical events: traumatic vs. neutral | PET | Britton et al. (2005) |
Visuo-verbal target detection: varying vs. fixed target conditions | PET | Clarck et al. (2003) |
Cued recollection of autobiographical events: traumatic vs. negative (nontraumatic) | fMRI | Driessen et al. (2004) |
Benign temperature vs. painful temperatures | fMRI | Geuze et al. (2007) |
Audiotaped script-driven autobiographical imagery: traumatic vs. Neutral | PET | Gilboa et al. (2004) |
Backward-masked images of combat vs. noncombat content | fMRI | Hendler et al. (2003) |
Traumatic vs. neutral pictures | fMRI | Hou et al. (2007) |
Fearful vs. neutral faces | fMRI | Kemp et al. (2009) |
Script-driven imagery of autobiographical traumatic event vs. rest | fMRI | Lanius et al. (2001) |
Script-driven imagery of autobiographical traumatic event vs. rest | fMRI | Lanius et al. (2002) |
1. Script-driven imagery of autobiographical traumatic event vs. rest 2. Script-driven imagery of autobiographical sad event vs. rest 3. Script-driven imagery of autobiographical anxious event vs. rest | fMRI | Lanius et al. (2003) |
Script-driven imagery of autobiographical traumatic event vs. rest | fMRI | Lanius et al. (2004) |
Connectivity: script-driven imagery of autobiographical traumatic event vs. rest | fMRI | Lanius et al. (2005) |
Trauma-related stimuli (combat sounds) vs. nonspecific arousing stimuli (white noise) | SPECT | Liberzon et al. (1999) |
Script-driven imagery of autobiographical events: traumatic vs. neutral | PET | Liberzon et al. (2003) |
Audiotaped script-driven imagery of autobiographical events: traumatic vs. neutral | SPECT | Lindauer et al. (2004) |
Script-driven imagery of autobiographical events: fearful vs. neutral | PET | Pardo et al. (2009) |
Trauma-related negative words vs. neutral words | fMRI | Protopopescu et al. (2005) |
Masked fearful faces vs. masked happy faces | fMRI | Rauch et al. (2000) |
Visual mental images of pictures: combat vs. neutral | PET | Shin et al. (1997) |
Audiotaped script-driven autobiographical imagery: traumatic vs. neutral | PET | Shin et al. (1999) |
Counting stroop: combat-related vs. neutral | fMRI | Shin et al. (2001) |
Audiotaped script-driven imagery of autobiographical events: traumatic vs. neutral | PET | Shin et al. (2004) |
Fearful vs. happy facial expressions | fMRI | Shin et al. (2005) |
Recognition: false alarms negative vs. baseline | fMRI | Thomaes et al. (2009) |
PET | Vermetten et al. (2007) | |
Emotional vs. neutral hits | fMRI | Whalley et al. (2009) |
Combat sounds vs. white noise | SPECT | Zubieta et al. (1999) |
Contrasts | Imaging modality | Study |
---|---|---|
Virtual Morris Water Task: cued navigation to a hidden ball vs. navigation to a visible ball | fMRI | Astur et al. (2006) |
Encoding of auditory paragraph vs. control condition (counting ‘d’s in the paragraph) | PET | Bremner et al. (2003) |
Neutral stroop vs. control stimulus | PET | Bremner et al. (2004) |
Target tones vs. standard tones | fMRI | Bryant et al. (2005) |
Rest | SPECT | Chung et al. (2006) |
Script-driven imagery of autobiographical neutral event vs. rest | fMRI | Lanius et al. (2004) |
Script-driven imagery of autobiographical neutral event vs. rest. | fMRI | Lanius et al. (2005) |
Rest | fMRI | Qingling et al. (2009) |
Rest | SPECT | Sachinvala et al. (2000) |
Auditory continuous performance task (tone volume discrimination) vs. rest | PET | Semple et al. (1996) |
Rest vs. auditory continuous performance task | PET | Semple et al. (2000) |
Neutral target words detection: varying vs. fixed target conditions | PET | Shaw et al. (2002) |
Recollection of neutral deeply vs. neutral shallowly encoded words | PET | Shin et al. (2004b) |
1. Encoding face-profession pairs (target stimuli) vs. watching face stimuli (control stimuli) 2. Retrieval of the matching-face profession vs. control task | fMRI | Werner et al. (2009) |
Old vs. new items recognition | fMRI | Whalley et al. (2009) |
Functional differences between PTSD and controls are often reported for the amygdala, but less so for the hippocampus. Thus, in structures that constitute the limbic core of the developmental trends, it appears that the functional aberrations in the paleocortical stream are more characteristic of PTSD pathology. Interestingly this pattern is similar for both emotional tasks where one might expect greater differences in amygdala activation, but also for the neutral contrasts (Fig. 10.2). Interestingly this pattern is the reverse of what was described for structural abnormalities. Importantly, however, outside the limbic core the picture is more consistent with the anatomical reports. The vast majority of reported differences in activation between PTSD and controls do not occur in the paleocortical stream, but rather in proisocortical and isocortical territories of the dorsal, archicortical, stream. These include regions in the posterior, dorsal and anterior cingulate, occipital and medial prefrontal cortices, as well as dorsolateral prefrontal cortex. By contrast, relatively few reports exist of differences in activation in ventral paleocortical regions outside the amygdala itself. The only other regions that seem to be relatively consistently implicated are the bilateral insula and left ventrolateral prefrontal cortex.
When stimuli and tasks are neutral (Table 10.2, Fig. 10.2) it is possible to observe several differences in the patterns of abnormal activations associated with a diagnosis of PTSD. First, there is even less overall consistency across studies than there is for emotional studies. This, however, is likely a function of the more varied tasks and conditions. It should be noted that there are many fewer studies that have used neutral stimuli than those that use emotional ones. With respect to patterns of abnormal activations, the rostral ACC and sub-callosal ACC do not show unique patterns of activity in response to neutral conditions. Only the dorsal ACC still appears to be recruited differently in PTSD when compared with controls. However, despite these specific differences between neutral and emotional stimuli, the same overall pattern can be detected, in that the majority of abnormal activations in PTSD appear to occur in the dorsal protogradation, and primarily in proisocortical and isocortical territories.
The overall pattern that arises from both types of functional neuroimaging studies nicely converges with the reports of structural abnormalities discussed above. Neuroimaging investigations appear to point to a primary abnormality of the dorsal archicortical stream, both structurally and functionally. The neurobiological basis of PTSD involves both core and later developed cortical territories of the archicortical protogradation. By contrast, the ventral paleocortical protogradation appears to display aberrant functional activity (but less structural alterations) of only the core structures (amygdala and to an extent insula).
Hypothetical Functional Significance
The observed patterns of functional irregularity may illuminate and help clarify the unique configurations of pathology and cognitive disruption associated with PTSD. Archicortical neocortical structures are broadly associated with the critical and complex task of multimodal integration of information. As such these structures support the representation and maintenance over time of the spatiotemporal context, in which events occur and behavior is executed. To facilitate such complex representations, dorsal systems perform functions such as (1) online processing of spatial cues and the location of objects in space; (2) allocation of spatial attention; (3) mnemonic functions that allow the flexible coding and retrieval of all forms of relationships among objects and events in both working memory and long-term memory. Accordingly, behavior mediated by the archicortical system can be characterized as “projectional”; that is, actions are derived from probabilistic models of the future based on previous experiences (Goldberg, 1985). These behaviors are controlled through the dorsal systems’ connection with dorsal sectors of the premotor cortex (see Figs. 10.1 and 10.2). Thus, the archicortical trend is responsible for coherence of both internal representations and motor behaviors, allowing for the creation and control of temporally and contextually bound and internally driven patterns of behavior and thought. They allow directed behavior to be organized over time, through support of working memory and extended planning processes. It is relatively straightforward to understand the characteristics of the re-experiencing and hyperarousal symptom clusters of PTSD in terms of dysfunction of archicortical neural structures. Contextually nonspecific “fight or flight” reactions, as well as difficulty maintaining attention, which are characteristic of hyperarousal, can result from aberrations from functional networks involving archicortical stream structures. Similarly, deficits in contextual fear conditioning leading to generalized nonspecific fear responses are known to result from damage to the hippocampus, the core structure of the archicortical stream. Finally, the fragmented, insulated nature of memory representations of traumatic memory can be a result of a dysfunction in systems whose role is to allow for coherent representations of time and space either at encoding or during retrieval and re-encoding.
On the other hand, paleocortical structures in the ventral stream code and respond to the motivational significance and the identity of external objects and stimuli. One of their most important functions is to support the integration of internally generated appetitive drives, with aversion, or attraction to external stimuli. The ventral system, through its extensive connections with and reliance on the amygdala and insular and temporal pole cortices, is well suited for integrating sensory information with viscera-autonomic responses. Thus it may serve an evaluative role in the processing of stimuli vis-à-vis their motivational significance in relation to internal states. One function the amygdala might carry out is parsing events or episodes out of the ongoing flow of ongoing experience. In doing so it instills visceroautonomic information into specific events, imbuing them with motivational significance, as part of the amygdala’s role in mediation of the fear response (Pribram, 1991). As such it may link motor sequences to stimuli in the environment in a responsive, segmented way (Goldberg, 1985), irrespective of the larger spatiotemporal context in which these events or stimuli occur. It is tempting to speculate that disruptions to these processes are at the heart of PTSD, because such characteristics appear to dominate the behavior of patients with PTSD. However, as noted above, aberrations of the ventral system both functionally and structurally are less consistently reported than those associated with the dorsal system. Moreover, there are indications that dorsal system abnormalities may constitute a risk factor for developing the disorder both structurally, as reflected by hippocampal volume (Gilbertson et al., 2002), and functionally as reflected by processing of spatial information (Gilbertson et al., 2007). Neurocognitive risk factors of PTSD also appear to point to greater involvement of dorsal system structures as reflected in deficits in visuo-spatial memory (Marx, Doron-Lamarca, Proctor, & Vasterling, 2009), working memory, attention, short-term memory, and even vocabulary achievement (Parslow & Jorm, 2007). Thus, one may cautiously hypothesize that if a primary deficit exists in PTSD it may be related to decreased ability to organize information and process it in a coherent and contextually bound manner. Observations of hyperactive amygdala may represent the normal function of this structure under conditions in which the archicortical moiety fails to perform its function, either during acute stress or chronically, but does not constitute a risk factor for developing the disorder (Milad et al., 2008).
Current models of PTSD emphasize abnormal hyperactivity of the amygdala on the one hand, but also hypoactivity of the medial prefrontal cortex. These canonical models highlight the role of medial aspects of the prefrontal cortex in extinction of conditioned fear, suggesting a combination of failed extinction and increased processing of fear stimuli are the definitive features of the disorder. This idea receives apparent support from elegant lesion and electrophysiological studies on rats that have demonstrated the infralimbic cortex in the rat is critical for extinction learning (Lebron, Milad, & Quirk, 2004; Milad & Quirk, 2002). Thus, studies that have found medial prefrontal cortex hypoactivation in patients with PTSD have asserted that it may reflect failed extinction processes in PTSD akin to the findings in the rat (Bremner, 2007; Liberzon & Sripada, 2008; Milad et al., 2006; Rauch et al., 2006; Shin et al., 2006).
While supported in fear conditioning studies of rodents, there is little direct evidence to support this model in humans. As described below, there are at least four reasons to treat such direct parallels between the animal and human research with caution. (1) The first reason is the uncertain homology between rodent and primate cortical cytoarchitectonic and functional organization, and the even more dubious relationship between functional imaging data in PTSD and the rat infralimbic cortex. With respect to rodent–primate cortical parallels, it has been argued by some that both sub-callosal ACC (BA 25) and rostral ACC (BA 32) in the primate, and by extension in humans, are homologous to the rat infralimbic cortex (Milad et al., 2006). However others have taken a more conservative approach and argued, just as convincingly, that the homology is restricted to sub-callosal (BA 25) and more ventral and caudal orbitofrontal parts of the primate brain (Barbas, 1995; Vertes, 2004) which include caudal BA 11 and 14 but not anterior ACC. Even if one accepts the former interpretation, and includes both areas 25 and 32, there is still a question with respect to the degree of correspondence between findings from neuroimaging studies of PTSD and the infralimbic cortex in the rat. In fact, fewer than 40 % of studies show abnormal activity in these regions, and some of these studies show hyper rather than hypo activations. (2) A second issue is the specificity of aberrant prefrontal activations associated with PTSD. This pertains to the question as to the extent to which presumed failed extinction is a core, fundamental deficit resulting in PTSD or just one of many dysfunctional processes, which might even be epiphenomenal to other core deficits. As can be seen in the current brief review, as well as in other more systematic meta-analyses (Etkin & Wager, 2007), Abnormal activations in areas that are considered homologous to the prelimbic region in rats (dorsal mPFC and DLPFC) are just as common, if not more frequent than those reported for areas 32 and 25. Moreover, even when medial prefrontal hypoactivations are observed, the center of activation is more rostral and dorsal, extending well beyond the proisocortical territory of the archicortical stream and into isocortical regions in Brodmann areas 9 and 10 (see Fig. 10.1 and cf. Fig. 5 in Milad et al., 2006). Opposite effects are reported in the rat with regard to prelimbic–amygdala interactions and their effect on extinction. These dorsal structures are likely more associated with higher-level processes of emotion regulation than with the biologically more basic process of extinction, although they might play a modulatory role in fear conditioning and extinction (see #4 below). (3) Thus the relationship between activation in PET and fMRI human neuroimaging studies and electrophysiological findings in animals is less than straightforward. It should be noted that the two types of studies have very different timescale: millisecond temporal resolution in the latter, and seconds to minutes in the former. Moreover, the relationship between cellular inhibition and excitation and hyper- or hypoactivation in neuroimaging is unknown. For example, both inhibitory and excitatory activity at the cellular level might appear as an increase in activity in neuroimaging, and hypoactivation in medial prefrontal cortex in PTSD could be interpreted either as lack of inhibition or as lack of excitatory input into other regions. (4) Finally, human neuroimaging studies have demonstrated extinction-related activity in area 25 (Phelps, Delgado, Nearing, & LeDoux, 2004), which appears to support the extinction failure model of PTSD. However, studies with healthy individuals have also demonstrated that more complex processes associated with emotion regulation critically mediate the contribution of these lower-level mechanisms (Delgado, Nearing, LeDoux, & Phelps, 2008; Schiller & Delgado, 2010). These high-level cognitive control processes are mediated by dorsal Medial and dorsolateral aspects of the PFC. As noted above abnormalities in activation of these regions are highly characteristic of PTSD and may reflect failure of emotion regulation processes, associated with deficient dorsal stream connectivity. These might be as critical a component in the evolution and persistence of PTSD as failed extinction and may in fact precede it.
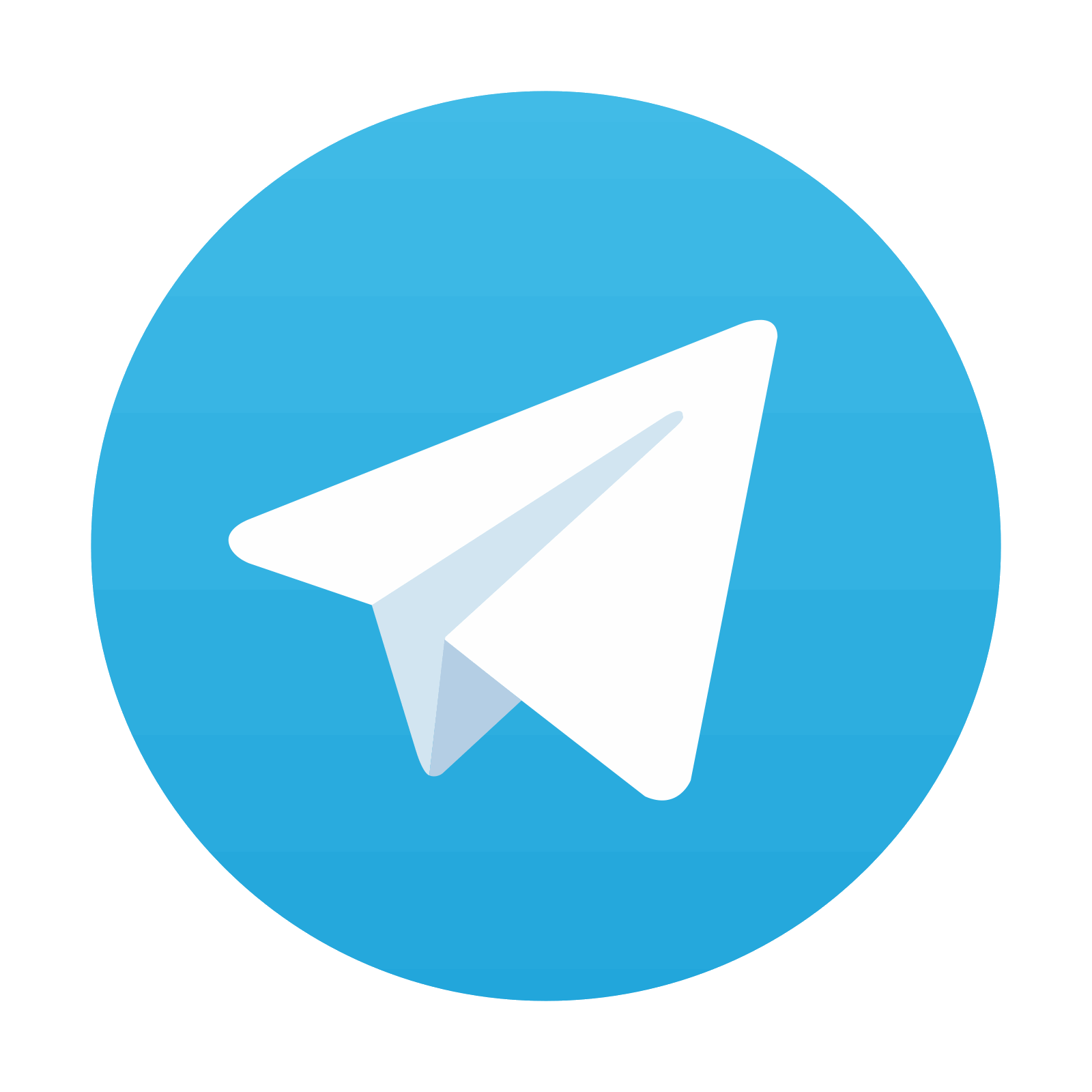
Stay updated, free articles. Join our Telegram channel
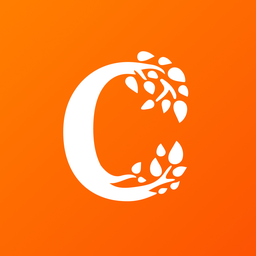
Full access? Get Clinical Tree
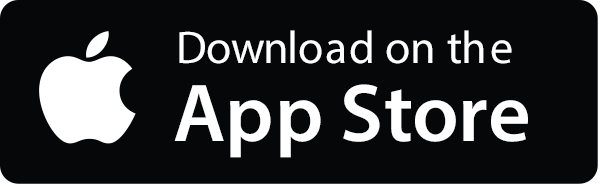
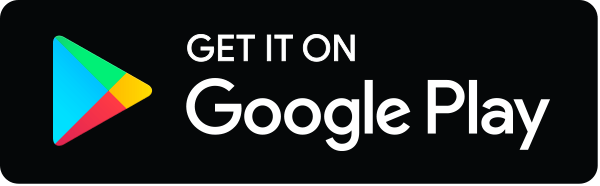