Careful consideration of functional lumbosacral anatomy reveals the capacity for pain generation in the disc, zygapophysial joint, sacroiliac joint, and surrounding ligaments. However, the methods used to definitively implicate a particular anatomic structure in axial low back pain have limitations. Anatomically and biomechanically, the discs and posterior elements are inextricably connected to a dynamic biotensegrity network of ligaments, muscles, and fascia. This article examines key lumbosacral anatomic structures and their functional interdependence at the macroscopic, microscopic, and biomechanical level. Particular attention is given to the capacity of each structure to generate low back pain.
Despite thorough clinical examinations and advances in diagnostic technology, the precise pain generator is difficult to identify in many patients with axial low back pain. The literature reveals significant limitations to the measures used to implicate a particular anatomic structure as an axial low back pain generator. The intervertebral disc, zygapophysial joint, and sacroiliac joint are believed to be common pain generators in axial low back pain, with prevalences reported as 5% to 39%, 15% to 40%, and 6% to 13%, respectively. Infection, malignancy, fracture, and inflammatory arthropathy are rare causes of low back pain. Recent research shows that a symptomatic hip joint commonly refers pain to the buttock. Most physical examination and imaging findings lack sensitivity and specificity. Degenerative changes of the lumbar spine visualized on magnetic resonance imaging (MRI) do not reliably predict low back pain. At present, our most precise estimates of prevalence of various pain generators are based on strict diagnostic criteria such as the dual block paradigm used for diagnosis of posterior element or sacroiliac pain. Provocation discography remains a controversial test.
Therapeutically, the individual presenting with acute or subacute axial low back pain is commonly offered a range of interventions including physical therapy, medication, functional restoration, acupuncture, chiropractic, epidural injections, or facet injections. Most individuals with low back pain have a favorable natural history and recover within a few weeks to months. In cases of severe or chronic pain, increasingly sophisticated treatment strategies have been developed based on a continually evolving understanding of lumbosacral anatomy and pathophysiology. Advanced imaging studies are often obtained to corroborate clinical findings and inform a variety of fluoroscopically guided diagnostic and therapeutic injections directed at the nerve roots, zygapophysial joints, or sacroiliac joints. Patients with severe, intractable axial low back pain often undergo provocative discography and may proceed to intradiscal injections, minimally invasive percutaneous decompression, or spinal fusion. When clinical findings and imaging identify a putative pain generator, a single, expertly performed invasive procedure and/or surgery may improve symptoms; however, relief is often partial or short lived.
The modest diagnostic and therapeutic success with low back pain may in part be the result of attempts to localize and target only one structure. Most medical and health professionals are still taught anatomy in a piecemeal fashion, studying one structure at a time rather than appreciating the spine as an integrated, interdependent, and dynamic biologic structure, suggesting that perhaps the focus on a single target may blind the physician to the big picture: the functional and pathophysiologic context of axial low back pain. The lumbosacral discs, posterior elements, and sacroiliac joint do not act in isolation; they function interdependently within a complex network of ligaments, muscles, nerves, and fascia via direct blending of tissue types. The low back is not a passive structure; it is dynamic and responsive to the environment via its specific connective tissue properties, biomechanical capacity, and sophisticated neurologic enneagrams. Diagnostic and therapeutic success in treating low back pain may ultimately lie in identifying dysfunction within this integrated network of structures rather than in searching for a single pain generator.
In addition to the visible physical connections among the discs, posterior elements of the spine, truncal muscles, and extremities, an increasing body of literature establishes that, at a cellular and biomechanical level, no anatomic structure acts in isolation. For example, multiple experimental studies in rat models have shown that, when a muscle’s extracellular matrix is subjected to mechanical force, the force is transmitted to the extracellular matrix of adjacent muscles, antagonist muscles, and to surrounding bony structures. Additional human studies have shown that force generation in a single human finger is coupled by force production in adjacent fingers, and that truncal muscles act synergistically during voluntary body sway.
Kinematically, the human spine’s capability for combined flexion, extension, twisting, and bearing weight from any direction is not consistent with its classic description as a pillar or column. The theory of biotensegrity (proposed by Stephen Levin ), grounded in cellular studies of surface tension and cytoskeletal structures, proposes support of the vertebral bodies by a highly organized continuous tension network consisting of muscles, ligaments, and fascia. In this system, the bones act as compression rods and the soft tissues as the tension elements. By this concept, a mechanical load encountered anywhere in the body is distributed through a continuous network of fascia, ligaments, and muscles suspending the entire skeleton, including the lumbar spine and sacrum. The membrane of a cell is another example of a structure with both elastic and rigid properties. The term biotensegrity was derived from the term tensegrity, or tensional integrity, first coined by Buckminster Fuller. This term was originally applied to artistic and architectural structures reflecting a balance between tension (cables) and compression (steel rods) elements. The original artistic inspiration is credited to Kenneth Snelson ( Figs. 1 and 2 ) for his classic Needle Tower which resembles a spine.
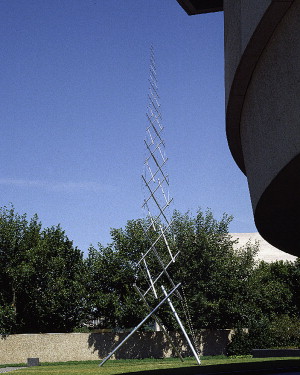
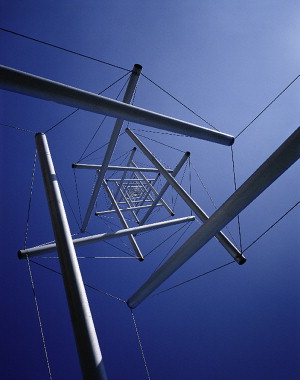
This article discusses lumbosacral anatomy and functional biomechanics and explores how these structures can malfunction in the setting of axial low back pain. Key anatomic structures and the functional interdependence of the elements at both the macroscopic and microscopic levels are discussed in detail. Lumbosacral anatomy and function are examined from a biotensegrity perspective.
Intervertebral disc
Disc Anatomy and Histology
The intervertebral disc is composed of a central nucleus pulposus surrounded by a tough annulus fibrosus ( Fig. 3 ). Within the annulus fibrosus, fibroblast cells continuously synthesize type I and II collagen into obliquely and perpendicularly oriented lamellar fibers, forming an overlapping pattern of near-complete circumferential rings. Lamellae are interconnected through a network of smaller fibers consisting of fibrillin, elastin, and aggrecan. The nucleus pulposus is composed of an extracellular matrix of type II collagen, proteoglycans, and noncollagenous proteins synthesized by chondrocytes to form a gelatinous inner core. The proteoglycans contain core proteins linked to chains of glycosaminoglycans and chains of hyaluronic acid. This matrix gives the nucleus pulposus its hydrostatic properties to allow for compression and bracing by the annulus fibrosus.
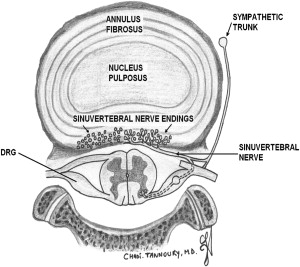
In a healthy disc, the extracellular matrix undergoes a balance of continual synthesis and degradation. Synthesis is primarily activated by fibroblast growth factor, transforming growth factor, and insulinlike growth factor, which inhibit the matrix metalloproteinases and allow for increased production of the matrix. Macrophages in the matrix secrete interleukin (IL)-1, interferon, and tumor necrosis factor (TNF)-α. These cytokines stimulate chondrocytes to secrete matrix metalloproteinases and facilitate degradation of the matrix. TNF-α in particular has been found to activate cell migration, increase vascular permeability, and reduce matrix synthesis in the nucleus pulposus. In a comparative study of cytokine expression, Lee and colleagues detected increased TNF-α and IL-8 in herniated disc tissue, and increased vascular endothelial growth factor and nerve growth factor in degenerated discs.
Pioneering anatomic studies of disc tissue revealed the inner annulus fibrosus and the nucleus pulposus to be avascular. Terminal vessels to the vertebral body endplates supply only the outer rings of the annulus fibrosus. Nutrition is believed to reach these areas via diffusion through the inner annulus fibers and extracellular matrix. In an early study of 4 cadavers (wherein history of low back pain was not reported), Bogduk and colleagues observed innervation of lumbar intervertebral discs by sinuvertebral nerve fibers on the outer posterior aspect of the annulus fibrosus. He hypothesized dual innervation from a sympathetic branch from the gray ramus communicans and a somatic branch from the ventral ramus. Subsequent immunohistochemistry studies indicate that the sympathetic pathway comprises most of the nerve fibers. Further anatomic studies revealed an extensive network of nerve fibers located on the disc surface and relatively few nerve endings, mainly unmyelinated and unencapsulated, within the annulus fibrosus itself. Nerve extension deep to the outer annulus is not seen in healthy discs.
Disc Biomechanics
Acting as a buttress and buffer between consecutive vertebral bodies, the intervertebral disc maintains structural integrity throughout a variety of movements. The annulus fibrosus provides both tensile and compression stiffness through its configuration of lamellar rings, and the semifluid nucleus pulposus can change its shape without losing volume. The nucleus pulposus transmits radial pressure on the vertebral endplates in response to a compressive load, which in turn is balanced by opposing tension within the annulus.
During forward sliding of vertebral bodies or application of shear force, the annulus resists slide via anteriorly and posteriorly oriented lamellar fibers. During flexion and extension, the corresponding anterior or posterior annulus compresses and stretches, resisting tension and pressure via deformation of the nucleus pulposus. Rotation causes circumferential movement of adjacent vertebral bodies, requiring that half of the lamellae separate and the other half approximate. Because only half of the fibers resist rotation, the annulus fibrosus may be more susceptible to rotational injury compared with compression injuries.
Disc Pathology
Disc degeneration is a state of histochemical dysfunction and gross structural changes of the annulus fibrosus and nucleus pulposus. Pain can be caused by obvious disc herniations and/or pathologic disc degeneration (vs normal aging). Disc herniation was first described by Dandy in 1929 and later by Mixter and Barr in 1934. Using oil-contrast myelography, Dandy described “loose cartilage simulating a tumor of the spinal cord.” During the herniated disc era, most axial and radicular low back pain was attributed to herniated discs; however, discography has shown that degenerated discs, particularly those with annular tears, were a significant cause of back pain. In 1980, Crock hypothesized that, following mechanical trauma, vertebral discs produce chemical substances that irritate the disc and nerve root, causing pain. Numerous subsequent studies have shown an imbalance between synthesis and degradation, resulting in histochemical changes in the extracellular matrix. As catabolic activity of matrix metalloproteinases, IL-1, and TNF-α increases, anabolic activity of insulinlike growth factor-1, transforming growth factor-β, and bone morphogenetic factors decreases. Consequently, the concentration of cells and matrix in the annulus fibrosus decreases. In addition, the proportion of collagen fiber types in the nucleus pulposus changes from type II to type I as the nucleus pulposus becomes desiccated and fibrotic. Declining nutrition, cell senescence, and the accumulation of degraded matrix products seem to contribute to this process.
Unlike healthy discs, degenerated discs develop pathologic innervation with extension of nerve fibers to the inner annulus and nucleus pulposus. Coppes and colleagues found that 8 of 10 degenerated disc specimens contained nerves within the inner annulus. Through nerve antibody testing in pathologic discs of patients with low back pain, Peng and colleagues detected both innervation and vascularization from the outer annulus fibrosus into the inner nucleus pulposus. Freemont and colleagues also detected nerve fibers in the inner annulus and nucleus pulposus tissue in degenerated discs of patients with low back pain, and found that these nerve fibers expressed substance P, a marker commonly associated with pain generation. Further studies have identified calcitonin gene–related peptide, also associated with pain generation, within the inner annulus and nucleus pulposus.
Mechanical factors have been implicated in disc degeneration. Disc degeneration occurs more commonly in the lower lumbar discs, discs immediately above a transitional vertebra, and discs adjacent to lumbar fusion. Several hypotheses involving mechanical changes at these levels are suggested. In vitro studies show that physiologic loading induces collagen formation, but excessive loading induces gene expression of matrix metalloproteinases, resulting in decreased gene expression of nearly all anabolic proteins. In cadaveric studies, acute torsion injuries are associated with tears in the posterior annulus similar to compression injury, whereas degenerative changes may cause tears in the inner annulus with extension into the periphery.
However, these studies have not been replicated in vivo. Animal studies of the association of compression with disc degeneration often have conflicting results, and human studies show that the intervertebral disc is able to withstand a wide range of compression forces during various activities of living. This finding is corroborated by an MRI study comparing elite equestrians with nonriding volunteers. Although the equestrian group had a higher rate of low back pain, there was no difference in disc degenerative findings between equestrians and age-matched controls. In a study of long-term effects of physical loading and exercise on back-related symptoms, elite weight lifters and soccer players showed greater degeneration throughout the lumbar spine compared with competitive runners and historically matched controls. Although imaging showed more degenerative findings, back pain was less common among athletes versus controls.
Biomechanical studies indicate that the vertebral endplate is more vulnerable to excessive compressive load than the intervertebral disc. Endplates can fracture in response to a sudden, large compressive load, or repeated large compressive loads. Endplate fractures are believed to increase interlaminar shear stress on the posterior annulus, resulting in depressurization such that the nucleus pulposus is unable to brace the annulus. The inner annulus then buckles anteriorly and the outer annulus buckles posteriorly, increasing the shear stress from compressive loads and thereby facilitating annular tears.
Conditions that reduce blood supply to the lumbar spine are associated with ischemic degenerative changes to the disc. Atheromatous lesions in the abdominal aorta and congenital hypoplasia of the lumbar arteries have been associated with disc degeneration. In addition, arteriolar constriction secondary to smoking has been associated with an increased incidence of disc herniation. When a vertebral fracture occurs, the resulting callus formation can occlude blood vessels providing nutrition to the disc, impairing maintenance of the matrix.
Although once attributed only to age-related changes, recent research has redefined the process of disc degeneration such that it can no longer be attributed to a singular process. Hadjipavlou and colleagues observe that “degeneration of the intervertebral disc can be defined as an age-dependent, cell-mediated molecular degradation process under genetic influence that is accelerated primarily by nutritional and mechanical factors, and secondly by toxic and metabolic factors.”
Clinical Correlates: Localization of Discogenic Pain Generators
Clinically, pain related to disc degeneration is currently distinguished from other sources of axial low back pain by first ruling out other pain generators (negative sacroiliac and facet joint blocks) and then proceeding to provocation discography. Provocation discography involves insertion of a small needle into the disc using fluoroscopic guidance, followed by pressure-, rate-, and volume-monitored instillation of contrast into the disc. The contrast provides hydraulic distension of the disc to determine whether it is chemically and/or mechanically sensitive. As a diagnostic test, provocative discography is controversial. In one study, Carragee and colleagues reported an unacceptably high (40%) false-positive rate for provocative discography in patients with chronic low back pain. Significant questions were raised about whether psychological comorbidity confounded provocative discography. In patients with somatization disorder, he reported a false-positive rate of 75%. However, a recent meta-analysis of false-positive rates for discography by Wolfer and colleagues showed a false-positive rate of less than 10% when the International Spine Interventional Society guidelines were followed.
Despite continuing controversy among stakeholders, discography remains the most reliable available test of the intervertebral disc as a pain generator. Schwarzer and colleagues used pain reproducible on provocation discography to assess the prevalence of disc disruption in 92 patients. Each patient was also required to have evidence of internal disc disruption on computed tomography (CT) and no reproduction of pain on testing of an additional disc. In this study, the prevalence of discogenic low back pain was calculated at 39%. When discogenic low back pain is suspected, clinical assessment includes identifying the location of pain and its relation to movements of the lumbar spine. There is wide variation in the sensitivity and specificity of the association between symptom location and positive discography. Identification of a directional preference, such as standing flexion, standing extension, supine flexion, prone extension, and rotation, is associated with positive provocation during discography, but two studies have indicated that these directional preferences are more specific than sensitive, and are not reliable.
In summary, the lumbar intervertebral disc contains a strong outer annulus fibrosus and a semifluid inner nucleus pulposus. Through the unique histochemistry of these two elements, the disc is designed to provide significant spinal support during a variety of movements, including forward slide, rotation, flexion, and extension. Numerous studies indicate that a significant subset of axial low back pain localizes to the intervertebral disc. However, disc degeneration is a complex biomechanical and histologic process that does not necessarily cause symptoms. The process by which some discs generate pain and others do not remains an area of active investigation.
Zygapophysial joints
Zygapophysial Joint Anatomy and Histology
Each lumbar spinal segment contains 2 posterolateral zygapophysial joints, often referred to colloquially as facet joints. Each zygapophysial joint consists of paired right and left inferior articular facets of one vertebra articulating with the paired superior articular facets of the vertebra below. The articular facets vary in shape and orientation to the sagittal plane. Superior articular facets may be flat or curved, containing either a C or J shape, and orientation may vary from 0 to 90 degrees based on level, with lower vertebrae oriented further from the sagittal plane. Zygapophysial joints have classic synovial joint features including hyaline cartilage, synovial membrane, and a joint capsule. The capsule is composed of 1 mm thick collagenous tissue located around the dorsal, superior, and inferior margins of the joint transversely connecting each articular process. The capsule contains 1 to 1.5 mL of fluid and consists of 2 layers: an outer layer of dense, parallel collagen fibers and an inner layer of irregular elastic fibers. Similarly to traditional synovial joints, a synovial lining lies deep to the fibrous capsule. Capsule fibers blend posteriorly with the multifidus muscle and anteriorly with the ligamentum flavum, which is continuous with the joint capsule.
The zygapophysial joint is innervated by 2 medial branches from consecutive vertebral levels. Each medial branch courses over the respective transverse process 1 level above its origination. The medial branch also innervates the multifidus muscle, the interspinous ligament, and the periosteum of the neural arch. One in 9 people may have variant innervation of the joint. Additional proposed innervation sources include the dorsal root ganglion and the paravertebral sympathetic ganglion ( Fig. 4 ).
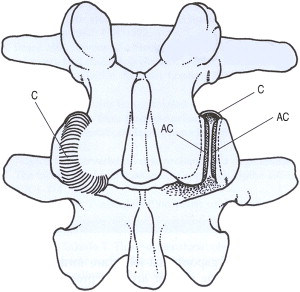
Zygapophysial Joint Biomechanics
Through its orientation and shape, the zygapophysial joint stabilizes the spine by limiting motion. The orientation of the joint determines its pattern of resistance. At the lower lumbar levels, zygapophysial joints contain greater surface area and are oriented more obliquely to provide more resistance to shear load and forward displacement; at higher lumbar levels, their sagittal orientation offers no resistance to forward displacement but strongly resists rotation. The shape of the joint provides additional resistance; C-shaped facets have increased surface area posteriorly and provide greater resistance to forward dislocation than J-shaped facets. Within the capsule, transverse orientation of fibers provides maximum resistance to flexion. With age, the zygapophysial joints weaken and change orientation from coronal to sagittal, providing less resistance against forward displacement.
Zygapophysial Joint Pathology
In 1911, Goldthwaite first suggested an association between facet joint variation and low back pain. In 1927, Putti dissected 75 cadavers and noted that degenerative changes in the facet joints were associated with sciatica. In 1933, Ghormley coined the term facet syndrome after finding similar symptoms in specimens subjected to sudden rotational strain. Although interest in the zygapophysial joint waned with increasing interest in the intervertebral disc, this joint has acquired significant attention in the last few decades.
Zygapophysial pathology is believed to occur through intimately linked direct and indirect processes. In the direct process, repetitive mechanical strain leads to osteoarthritic changes within the joint, including subchondral sclerosis and cartilage degeneration. This in turn leads to the indirect process, including zygapophysial hypertrophy and cystic formation of the synovial membrane. This cystic formation occurs most commonly at the L4 to L5 level.
On review of autopsy studies describing zygapophysial joints, Uhrenholt and colleagues observed subchondral sclerosis, cartilage fibrillation and splitting, and thinning of the articular surface in degenerated joints. In samples taken from a series of patients undergoing lumbar spinal fusion, histologic changes similar to chondromalacia patellae and osteoarthritis of large joints were visualized, including cartilage necrosis and exposure of subchondral bone. Similar changes were visualized in patients who underwent repetitive strain patterns. In response to repetitive strain, these joints can fill with fluid, distend, and cause pain likely related to capsular stretch. Capsular stretch is also associated with reflex spasm of the erector spinae and multifidus muscles.
Facet tropism is defined as asymmetry between the right and left facet orientation and has also previously been implicated as a pain generator. Loss of coronal orientation is seen with age in 20% to 40% of the population. This change in orientation was strongly associated with osteoarthritis in a study of 111 patients by Fujiwara and colleagues. A more recent study involving 188 patients in the Framingham Heart Study showed that sagittal orientation of the joint was only associated with osteoarthritis at the L4 to L5 level, but neither study reflected an association between facet tropism and zygapophysial osteoarthritis.
Because the zygapophysial joint is richly innervated, these degenerative changes could result in pain. Recent findings of substance P and calcitonin gene–related peptide within the capsule suggest its capacity to serve as a pain generator. Phospholipase A2 has also been implicated as an inflammatory mediator within the zygapophysial joint, increasing inflammation and edema and causing prolonged nociceptive excitation.
Clinical Correlates: Localization of Zygapophysial Joint Pain Generators
The prevalence of zygapophysial joint pain is hypothesized to range between 15% and 40%. Diagnosis of lumbar back pain secondary to zygapophysial joint degenerative changes is often challenging. Most clinicians rely on several history-related characteristics of the pain, including unilateral pain worse with extension, and pain slightly lateral to the midline. Clinicians may then confirm possible causes using imaging consistent with facet degeneration. However, currently there is no evidence to suggest that any specific history, physical examination findings, or imaging findings will predict response to a medial branch block.
Revel and colleagues created 7 diagnostic criteria for identifying zygapophysial joint pain: age more than 65 years; pain relieved by recumbent position; no exacerbation with cough, flexion, or extension; no pain with rising from flexion; and the extension-rotation test. He reported that 92% of patients having 5 of 7 criteria responded to a medial branch block. However, in a subsequent study, these results were not replicated; and the 7 criteria exhibited specificity near 90% and a sensitivity of only 17%.
In a series of 192 patients who reported positive response to a medial branch block, Cohen and colleagues studied multiple factors believed to predict this outcome, including duration of pain, MRI abnormalities, and levels treated. The only factor associated with a successful medial branch block was paraspinal tenderness. A prospective statistical study showed no correlation between radiographic evidence of zygapophysial joint degeneration and response to a medial branch block. More recently, MRI with sagittal short-tau inversion recovery (STIR) seems promising. In a study of serial STIR imaging of axial low back pain in patients with visible zygapophysial joint edema, patients who noted change in their pain score were also noted to have changes in the intensity of edema at the zygapophysial joint.
In summary, the zygapophysial joint connects the superior articular facet of each consecutive vertebra with the inferior articular facet of the vertebra above. These true synovial joints are particularly active in resisting flexion and forward slide of the vertebrae. With age and trauma, these joints can exhibit pathologic changes similar to those in seen large joint osteoarthritis. Pain generation in the zygapophysial joint may result from osteoarthritic changes and altered orientation of the joint in space. The current state of the art for diagnosis of zygapophysial joint pain uses fluoroscopically guided comparative medial branch blocks.
Zygapophysial joints
Zygapophysial Joint Anatomy and Histology
Each lumbar spinal segment contains 2 posterolateral zygapophysial joints, often referred to colloquially as facet joints. Each zygapophysial joint consists of paired right and left inferior articular facets of one vertebra articulating with the paired superior articular facets of the vertebra below. The articular facets vary in shape and orientation to the sagittal plane. Superior articular facets may be flat or curved, containing either a C or J shape, and orientation may vary from 0 to 90 degrees based on level, with lower vertebrae oriented further from the sagittal plane. Zygapophysial joints have classic synovial joint features including hyaline cartilage, synovial membrane, and a joint capsule. The capsule is composed of 1 mm thick collagenous tissue located around the dorsal, superior, and inferior margins of the joint transversely connecting each articular process. The capsule contains 1 to 1.5 mL of fluid and consists of 2 layers: an outer layer of dense, parallel collagen fibers and an inner layer of irregular elastic fibers. Similarly to traditional synovial joints, a synovial lining lies deep to the fibrous capsule. Capsule fibers blend posteriorly with the multifidus muscle and anteriorly with the ligamentum flavum, which is continuous with the joint capsule.
The zygapophysial joint is innervated by 2 medial branches from consecutive vertebral levels. Each medial branch courses over the respective transverse process 1 level above its origination. The medial branch also innervates the multifidus muscle, the interspinous ligament, and the periosteum of the neural arch. One in 9 people may have variant innervation of the joint. Additional proposed innervation sources include the dorsal root ganglion and the paravertebral sympathetic ganglion ( Fig. 4 ).
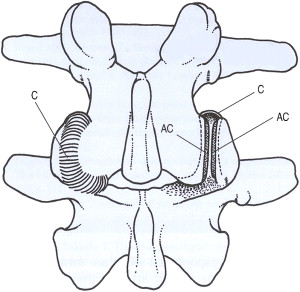
Zygapophysial Joint Biomechanics
Through its orientation and shape, the zygapophysial joint stabilizes the spine by limiting motion. The orientation of the joint determines its pattern of resistance. At the lower lumbar levels, zygapophysial joints contain greater surface area and are oriented more obliquely to provide more resistance to shear load and forward displacement; at higher lumbar levels, their sagittal orientation offers no resistance to forward displacement but strongly resists rotation. The shape of the joint provides additional resistance; C-shaped facets have increased surface area posteriorly and provide greater resistance to forward dislocation than J-shaped facets. Within the capsule, transverse orientation of fibers provides maximum resistance to flexion. With age, the zygapophysial joints weaken and change orientation from coronal to sagittal, providing less resistance against forward displacement.
Zygapophysial Joint Pathology
In 1911, Goldthwaite first suggested an association between facet joint variation and low back pain. In 1927, Putti dissected 75 cadavers and noted that degenerative changes in the facet joints were associated with sciatica. In 1933, Ghormley coined the term facet syndrome after finding similar symptoms in specimens subjected to sudden rotational strain. Although interest in the zygapophysial joint waned with increasing interest in the intervertebral disc, this joint has acquired significant attention in the last few decades.
Zygapophysial pathology is believed to occur through intimately linked direct and indirect processes. In the direct process, repetitive mechanical strain leads to osteoarthritic changes within the joint, including subchondral sclerosis and cartilage degeneration. This in turn leads to the indirect process, including zygapophysial hypertrophy and cystic formation of the synovial membrane. This cystic formation occurs most commonly at the L4 to L5 level.
On review of autopsy studies describing zygapophysial joints, Uhrenholt and colleagues observed subchondral sclerosis, cartilage fibrillation and splitting, and thinning of the articular surface in degenerated joints. In samples taken from a series of patients undergoing lumbar spinal fusion, histologic changes similar to chondromalacia patellae and osteoarthritis of large joints were visualized, including cartilage necrosis and exposure of subchondral bone. Similar changes were visualized in patients who underwent repetitive strain patterns. In response to repetitive strain, these joints can fill with fluid, distend, and cause pain likely related to capsular stretch. Capsular stretch is also associated with reflex spasm of the erector spinae and multifidus muscles.
Facet tropism is defined as asymmetry between the right and left facet orientation and has also previously been implicated as a pain generator. Loss of coronal orientation is seen with age in 20% to 40% of the population. This change in orientation was strongly associated with osteoarthritis in a study of 111 patients by Fujiwara and colleagues. A more recent study involving 188 patients in the Framingham Heart Study showed that sagittal orientation of the joint was only associated with osteoarthritis at the L4 to L5 level, but neither study reflected an association between facet tropism and zygapophysial osteoarthritis.
Because the zygapophysial joint is richly innervated, these degenerative changes could result in pain. Recent findings of substance P and calcitonin gene–related peptide within the capsule suggest its capacity to serve as a pain generator. Phospholipase A2 has also been implicated as an inflammatory mediator within the zygapophysial joint, increasing inflammation and edema and causing prolonged nociceptive excitation.
Clinical Correlates: Localization of Zygapophysial Joint Pain Generators
The prevalence of zygapophysial joint pain is hypothesized to range between 15% and 40%. Diagnosis of lumbar back pain secondary to zygapophysial joint degenerative changes is often challenging. Most clinicians rely on several history-related characteristics of the pain, including unilateral pain worse with extension, and pain slightly lateral to the midline. Clinicians may then confirm possible causes using imaging consistent with facet degeneration. However, currently there is no evidence to suggest that any specific history, physical examination findings, or imaging findings will predict response to a medial branch block.
Revel and colleagues created 7 diagnostic criteria for identifying zygapophysial joint pain: age more than 65 years; pain relieved by recumbent position; no exacerbation with cough, flexion, or extension; no pain with rising from flexion; and the extension-rotation test. He reported that 92% of patients having 5 of 7 criteria responded to a medial branch block. However, in a subsequent study, these results were not replicated; and the 7 criteria exhibited specificity near 90% and a sensitivity of only 17%.
In a series of 192 patients who reported positive response to a medial branch block, Cohen and colleagues studied multiple factors believed to predict this outcome, including duration of pain, MRI abnormalities, and levels treated. The only factor associated with a successful medial branch block was paraspinal tenderness. A prospective statistical study showed no correlation between radiographic evidence of zygapophysial joint degeneration and response to a medial branch block. More recently, MRI with sagittal short-tau inversion recovery (STIR) seems promising. In a study of serial STIR imaging of axial low back pain in patients with visible zygapophysial joint edema, patients who noted change in their pain score were also noted to have changes in the intensity of edema at the zygapophysial joint.
In summary, the zygapophysial joint connects the superior articular facet of each consecutive vertebra with the inferior articular facet of the vertebra above. These true synovial joints are particularly active in resisting flexion and forward slide of the vertebrae. With age and trauma, these joints can exhibit pathologic changes similar to those in seen large joint osteoarthritis. Pain generation in the zygapophysial joint may result from osteoarthritic changes and altered orientation of the joint in space. The current state of the art for diagnosis of zygapophysial joint pain uses fluoroscopically guided comparative medial branch blocks.
The sacroiliac joint/ligament complex
Sacroiliac Anatomy
The sacroiliac joint connects the spine and the pelvis through a diarthrodial joint between 2 bony surfaces: the sacrum and ilium. The sacrum is wedged between 2 iliac bones to form the posterior wall of the pelvis. The space between the concave sacrum and convex ilium is approximately 1 to 2 mm wide, and the joint maintains a C shape with convexity directed anteriorly and inferiorly. The joint surfaces are lined with 1 mm of hyaline cartilage at the iliac end and 6 mm at the sacral end. The superior portion of the joint has the histologic qualities of a symphysis and is attached to a dense network of surrounding stabilizing ligaments, whereas the inferior portion of the joint has the histologic characteristics of a synovial joint.
Several strong ligaments maintain the integrity, shape, and position of the sacroiliac joint. The dense interosseous fibers constitute the thickest connections between the sacrum and the ilium. The anterior and posterior sacroiliac ligaments strengthen this connection on their respective aspects of the sacrum and ilium ( Fig. 5 ). The ligaments and the joint are so closely connected that the anterior sacroiliac ligament is a continuation of the anterior capsule. The sacrotuberous ligament attaches at the sacroiliac joint capsule, the coccygeal vertebrae, and the ischial tuberosity. Sacrotuberous fibers also blend with the biceps femoris tendon distally, and the deep multifidus and piriformis proximally. Caudal traction on the long head of the biceps femoris increases tension in the sacrotuberous ligament. The sacrospinous ligament attaches at the sacroiliac joint capsule, the lower sacral and coccygeal vertebrae, and the ischial spine.
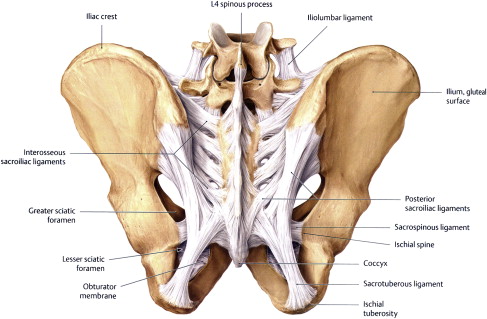
The innervation of the sacroiliac joint is under debate. Mechanoreceptors and nerve fascicles have been observed in the sacroiliac joint with gold chloride staining. Further histologic studies verify the presence of innervation to the joint capsule and the ligaments. Nerve fascicles containing myelinated fibers, unmyelinated fibers, paciniform mechanoreceptors, and nonpaciniform mechanoreceptors have been identified, suggesting that both pain and proprioception are transmitted through the sacroiliac joint. However, the source of these nerves is controversial. Based on cadaveric studies, Fortin proposes that the joint receives exclusive innervations from the sacral S1 to S4 dorsal rami. Electrical and mechanical stimulation studies suggest that the innervation arises predominantly via the L4 to S1 nerve root, with secondary contribution from the superior gluteal nerve.
Sacroiliac Biomechanics
The sacroiliac joint transmits vertical forces through the spine, the pelvis, and the extremities. Its C shape, with interlocking lever arms at S2 and numerous ridges and depressions, is believed to increase stability. During activity, this joint allows 2 to 3 degrees of rotation and 0.5 to 1.6 mm of translation. The axis of motion crosses the pelvis obliquely. During hip flexion, the ipsilateral ilium moves posteriorly and caudally, compressing the sacrum and pivoting at the pubic symphysis. During hip extension, the ipsilateral ilium moves anteriorly and away from the sacrum. This oblique plane of movement is consistent with 3 dimensional movement of the pelvis required during ambulation.
The thick interosseous sacroiliac ligament intimately connects the sacrum and ilium and is believed to secure the bony interlocking mechanism. In addition to strengthening the interosseous ligament, the anterior and posterior sacroiliac ligaments prevent forward rocking (nutation) and backward rocking (counternutation) of the pelvis, respectively. The sacrospinous and sacrotuberous ligaments prevent rotation of the caudal end of the sacrum. Although the sacroiliac ligament attaches to multifidus fibers directly and to additional muscles via the sacrotuberous ligament, the joint’s movement is minimal. Rotational axis studies by Lavignolle and colleagues suggest that the sacroiliac joint’s primary function is absorption of mechanical force from the lower limbs across the pelvic girdle.
Sacroiliac Pathology
Mechanical changes, inflammation, trauma, and degeneration all occur within the sacroiliac joint, and each can be associated with pain generation. Anatomically, the joint can undergo significant changes secondary to osteoarthritis or repetitive strain patterns, including capsular or synovial disruption, chondromalacia, and cartilage destruction. In a study by Chou and colleagues, the most likely cause of sacroiliac joint pain confirmed by injection was trauma in 44% of patients, idiopathic in 35% of patients, and repeated stress in 21% of patients.
The extra-articular ligaments and muscles may contribute to sacroiliac joint pathology through hypomobility or hypermobility, abnormal joint mechanics, soft tissue injury, and inflammation. Sacroiliac ligaments also seem to be significant pain generators. In a comparative study, Murakami and colleagues found that 36% of patients receiving intraarticular injections alone experienced relief of low back pain, in contrast to 92% of patients receiving extra-articular injections.
Biomechanical and muscle length discrepancies may predispose a patient to sacroiliac pain secondary to altered gait patterns and repetitive stress on the joint. Fortin and Falco hypothesized that an imbalanced unilateral load changes the balance of the C-shaped joint and increases sacral rotation when 1 ilia is fixed and the other is rotationally loaded. They identified biomechanical dysfunction of the sacroiliac joint in athletes who participate in unidirectional repetitive activities, including forward displacement and rotation. Pregnancy also predisposes to sacroiliac pain through weight gain, lordotic posturing, and hormone-induced ligamentous laxity.
Clinical Correlates: Localization of Sacroiliac Pain Generators
Overall, clinical tests for sacroiliac pain have shown much better sensitivity and specificity than those for disc pain or facet pain. Clinical tests used to assess for sacroiliac pain generators include the Faber test, distraction/compression, focal joint tenderness, the Gillett femoral shear test, and the modified Gaenslen test. Young and colleagues found that the likelihood of low back pain arising from the sacroiliac joint was significantly higher if 3 or more of these tests are positive and if the pain is unilateral below L5 without lumbar pain. However, in a study of 12 physical examination findings individually and in combination, Dreyfuss and colleagues found that none could predict intraarticular sacroiliac joint pain as determined by a single positive block with more than 90% pain relief. A recent systematic review validated the thigh thrust test and sacroiliac compression test as diagnostic indicators of sacroiliac joint pain, finding that, if 3 of 5 provocation tests including compression, distraction, thigh thrust, Gaenslen test, and Patrick sign were positive, the specificity reached 100% and the sensitivity was 77% to 87%. Several small studies have shown a positive correlation between successful sacroiliac joint block and clinical localization of primary pain to the Fortin point, which lies within 2 cm of the posterior superior iliac crest.
In the last decade, a newer functional physical examination test, called the active straight leg raise (ASLR), has been used to assess pelvic load transfer in patients with sacroiliac or posterior pelvic girdle pain. Lying supine, with legs extended, the patient is asked to raise the heel approximately 30 cm off the table. A positive test is described if the patient subjectively reports one leg to be heavier than the other. The ASLR test reveals that sacroiliac joint pain can cause global dysfunction of lumbopelvic motor control patterns and respiration in low load conditions. O’Sullivan and colleagues found that subjects with sacroiliac joint pain combined with positive ASLR test had increased pelvic floor descent, decreased diaphragmatic excursion, and increased minute ventilation. With manual compression of the ilia (similar to using a sacroiliac belt), participants with sacroiliac pain exhibited neuromuscular patterns normalized to those of matched controls.
Imaging is of limited diagnostic value, with CT sensitivity of 57% and specificity of 69% in predicting positive response to intraarticular sacroiliac injection. Specificity of bone scans has been calculated to be as high as 90%, but with only 46% sensitivity in predicting positive response to intraarticular sacroiliac block. Imaging is most useful when there is suspicion for a fracture, infection, or malignant or rheumatologic cause of sacroiliac joint pain.
In summary, the sacroiliac joint binds the spine to the pelvis and plays a critical role in force transmission to the extremities. Given its unique position, this joint and its supportive ligaments and muscles provide minimal amounts of rotation and translation on an oblique axis to allow a variety of pelvic movements during stance and ambulation. The sacroiliac joint is also believed to be intimately involved in core stability, including pelvic floor function. Increased success of periarticular, compared with intraarticular, injections indicates that sacroiliac joint pain is not confined to the joint capsule alone but also can arise from with well-innervated contiguous ligaments and muscle fibers.
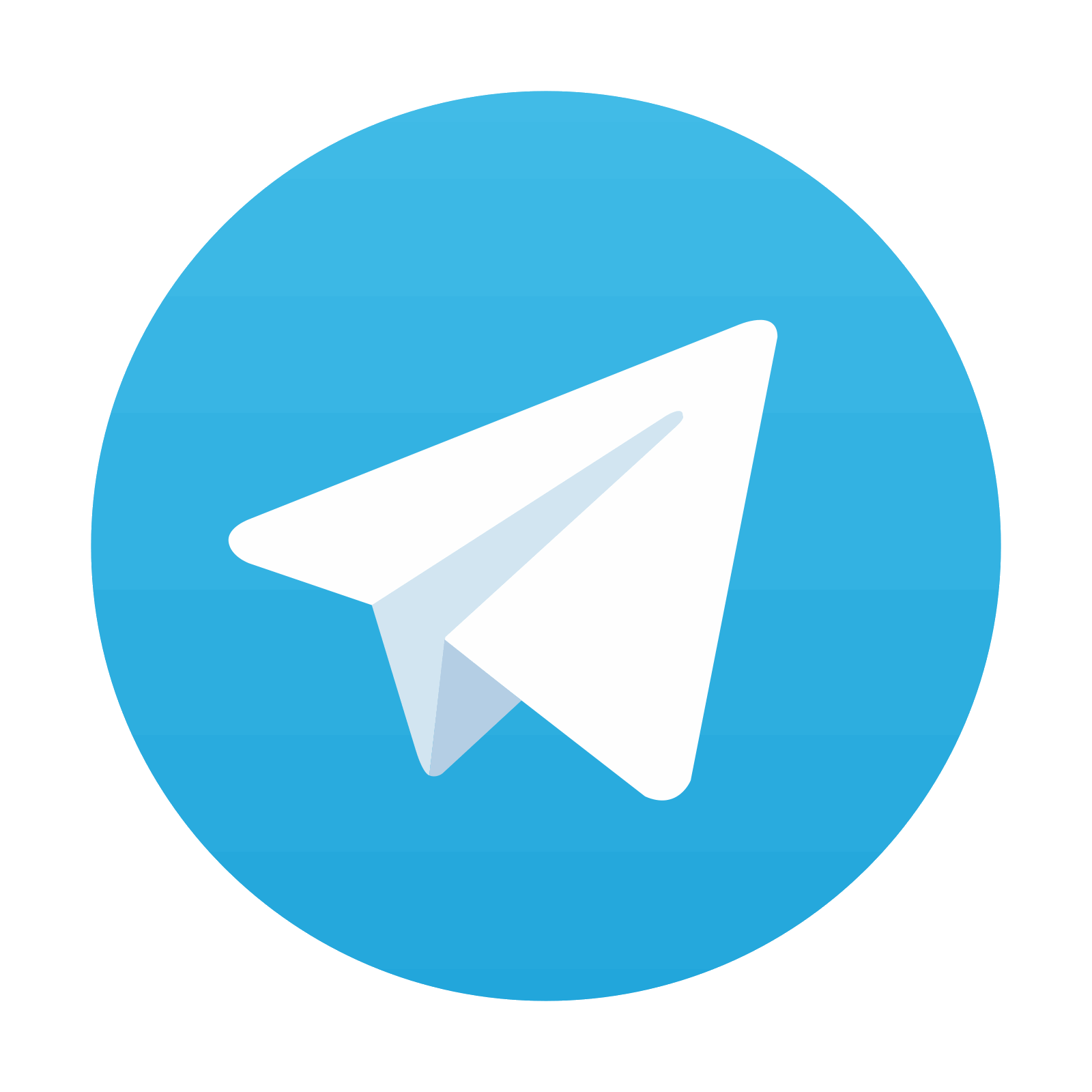
Stay updated, free articles. Join our Telegram channel
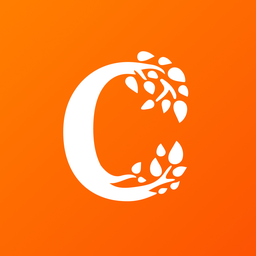
Full access? Get Clinical Tree
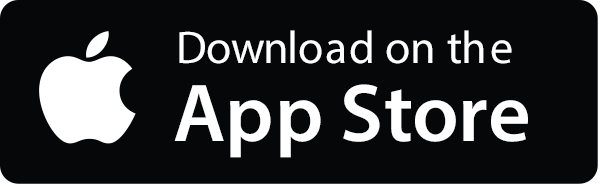
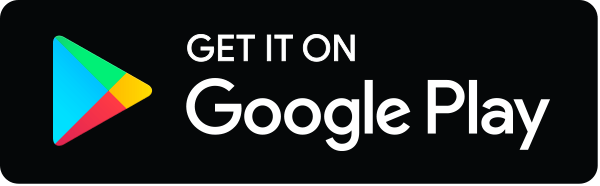