Form and Function of Skeletal Muscle
Johnny Huard, PhD
Hajime Utsunomiya, MD, PhD
Sudheer K. Ravuri, PhD
Marc J. Philippon, MD
Dr. Huard or an immediate family member serves as a board member, owner, officer, or committee member of ORS and Orthopaedic Research Society. Dr. Philippon or an immediate family member has received royalties from Arthrex, Arthrosurface, Bledsoe, CONMED Linvatec, and DonJoy; serves as a paid consultant to or is an employee of Smith & Nephew, MIS; has stock or stock options held in Arthrosurface, MIS, MJP Innovations, LLC, and Vail Valley Surgery Center; has received research or institutional support from Smith and Nephew, Ossur, Arthrex, Siemens, and Vail Vally Medical Center; has received nonincome support (such as equipment or services), commercially derived honoraria, or other non-research-related funding (such as paid travel) from Smith & Nephew; and serves as a board member, owner, officer, or committee member of ASIAM, the International Society for Hip Arthroscopy, the American Orthopaedic Society for Sports Medicine, the Steadman Philippon Research Institute, the Vail Health Services, and the Vail Valley Surgery Center Governing Council. Neither of the following authors nor any immediate family member has received anything of value from or has stock or stock options held in a commercial company or institution related directly or indirectly to the subject of this chapter: Dr. Utsunomiya and Dr. Ravuri.
Keywords: atrophy; extracellular matrix; fibrosis; losartan; musculoskeletal tissue; myofibrils; PRP; stem cells
INTRODUCTION
Muscle injuries are extremely common, and they tend to recur. The intrinsic ability of the skeletal muscle to repair after injury because of the activation of muscle progenitor cells often leads to the development of fibrotic tissue at the original site of injury. Fibrotic tissue consequently limits force production and predisposes the muscle to reinjury. This chapter reviews the basic anatomy and physiology of skeletal muscle, the basic mechanisms of muscle growth and adaptation, the types of muscle injuries, and the stages of the healing process. Recent achievements in biological approaches to improve muscle healing after injury include methods for enhancing muscle regeneration and reducing muscle fibrosis. Other useful and less studied strategies to enhance skeletal muscle repair include gene therapy, exercise, neuromuscular electrical stimulation, blood flow restriction, and, potentially, massage therapy. It is clear that basic science and preclinical studies must be performed to ensure the safety of such therapies before they can become part of standard orthopaedic care.
TYPES OF SKELETAL MUSCLE
Skeletal muscles can be categorized based on the distribution of myofibers and their insertion into the tendons. Muscles with parallel fibers are most common. These parallel muscles can produce a substantial change in length with relatively little force, but their relatively small cross-sectional area means that total force production is relatively small. A unipennate muscle has fibers that insert on only one side of a tendon, thus creating a relatively large cross section and greater strength but less change in length. Fibers in a bipennate muscle consolidate on two sides of the inserting tendon, increasing relative cross section, allowing greater strength than a unipennate muscle, with smaller changes in length.
THE STRUCTURE OF SKELETAL MUSCLE
GROSS STRUCTURE
Skeletal muscle is enclosed by fascia (a smooth sheath of connective tissue that allows the muscle to slide past surrounding tissues). A muscle is composed of bundles of muscle fibers called fascicles, which are connected by a delicate connective tissue called epimysium. Fascicles are composed of hundreds of muscle fibers enclosed by endomysium, and each individual fiber (myofiber) is enveloped by perimysium. Each myofiber is a long, thin, tubular structure, often many centimeters long and usually running the entire length of the muscle.
CELL STRUCTURE
The skeletal muscle cell has a plasma membrane called the sarcolemma, which is surrounded by several layers of tissue including a connective tissue basement membrane, a reticular layer, and the extracellular matrix. These surrounding layers make up the endomysium. The skeletal muscle fiber contains multiple nuclei, typically situated at the periphery of the cell just beneath the sarcolemma. The unique structure of the sarcolemma makes the muscle fiber an efficient contractile apparatus. The sarcolemma extends into the cell, surrounding the contractile elements of the muscle cell (myofibrils) and forming transverse tubules. Smaller longitudinal tubules extend from the transverse tubules to interact with the sarcoplasmic reticulum, which is a system of membrane-bound organelles that stores calcium. The sarcoplasmic reticulum contains several enzymes to regulate calcium sequestering and release; the intracellular concentration of calcium turns the contractile apparatus on and off. The permeating design of the sarcolemma and sarcoplasmic reticulum increases the cross-sectional area, permits quick transmission of action potentials to the contractile elements, and provides a reservoir of calcium ions close to the contraction.
SARCOMERES
The myofibrils are a highly ordered set of proteins arranged in parallel along the axis of the cell. This arrangement ensures a cumulative effect of contractions. A distinct banding pattern produces the characteristic striated appearance of alternating light and dark bands seen under the microscope. Myofibrils are divided into contractile units called sarcomeres. The dark band (the A band) represents thick filaments centered around interconnecting proteins (the M line). The thick filaments are primarily composed of myosin, which is a large protein made up of a long, insoluble helical portion and two globular portions called cross-bridges. The globular portions are capable of binding the thin filaments and hydrolyzing high-energy adenosine triphosphate (ATP) to release stored chemical energy. The thick filaments also contain C protein, M protein, myosin, titin, and creatine kinase.
The thin filaments attach to an interconnecting set of structural proteins (the Z line) and extend into the middle of the sarcomere. The thin filaments are primarily composed of the protein actin as well as troponin and tropomyosin. Additional proteins at the Z line include desmin, α-actinin, and filamin. The overlap of actin and myosin allows binding between the proteins and subsequent contraction of the myofibrils.
MUSCLE CONTRACTION
Muscular contraction is produced by interaction between the thick and thin filaments. The myosin molecules in the thick filaments form cross-bridges with the actin molecules of the thin filaments. The globular domain of the myosin molecule hydrolyzes ATP to adenosine diphosphate, causing a conformational change in the myosin molecule that pushes the filaments past each other. The myosin molecule quickly releases the actin molecule and returns to its original conformation. This ATP-dependent cycle repeats in rapid succession to cause the thick and thin filaments to pull toward each other. Because of the relative polarities of the thick and thin filaments, active cross-bridging results only in a sliding of the thick and filament complexes toward each other and a shortening or resistance to stretching of the sarcomere.
Cross-bridge cycling is modulated by intracellular calcium through the regulatory proteins troponin and tropomyosin. Tropomyosin is a molecule on the thin filament that prevents myosin-actin interaction. Troponin is a calcium-dependent regulatory protein with three domains: the troponin-C subunit binds calcium, troponin-T binds tropomyosin, and troponin-I is inhibitory to the actin-myosin interaction. When troponin-C binds calcium, the molecule changes conformation, relieving the troponin-I and tropomyosin inhibition of myosin-actin cross-bridging. Therefore, when intracellular calcium levels are high, cross-bridge cycling is active, and the myofibrils contract.
Each myofibril is stimulated by one nerve axon, but one axon can branch and stimulate multiple myofibrils. All of the myofibrils contacted by one axon constitute a motor unit. When the motor unit is activated, its entire muscle fibers contract. The number of muscle fibers in a motor unit ranges from approximately 10 to 1,000; in general, muscles that require fine motor control have relatively few fibers per motor unit. The neuromuscular junction is the site at which the nervous system controls muscular contraction. The neuromuscular junction is composed of the presynaptic axon, the synaptic cleft, and the postsynaptic muscle cell.
During the conversion of neuronal depolarization to muscular contraction, an action potential from the motor neuron reaches the axon terminal, releasing acetylcholine into the synaptic cleft. Acetylcholine binds to postsynaptic receptors, which respond by increasing permeability to sodium and depolarizing the sarcolemma. This depolarization spreads through the transverse tubules, stimulating the sarcoplasmic reticulum to release calcium into the cell. Calcium binds to the
protein troponin, allowing myosin filaments to slide past actin filaments in an ATP-dependent process and shorten the muscle fiber. Contraction ceases when acetylcholine is degraded at the postsynaptic receptor, the membrane repolarizes, and calcium is pumped back into the sarcoplasmic reticulum.
protein troponin, allowing myosin filaments to slide past actin filaments in an ATP-dependent process and shorten the muscle fiber. Contraction ceases when acetylcholine is degraded at the postsynaptic receptor, the membrane repolarizes, and calcium is pumped back into the sarcoplasmic reticulum.
A single action potential results in a quick contraction followed by relaxation. The length of the contraction ranges from approximately 7.5 ms (for fast myofibers) to 100 ms (for slow myofibers). Unlike nerve tissue, muscle tissue has no refractory period for the contractile mechanism; a rapid sequence of depolarizations results in a continuous, tetanic contraction.
TYPES OF CONTRACTIONS
Muscular contractions typically are described as shortening the muscle in a concentric contraction. Several other types of contractions also exist. In an eccentric contraction, myosin-actin cross-bridges are formed, but the opposing force is greater than the force created by the muscle and the muscle therefore lengthens. In an isometric contraction, the force of contraction equals the opposing force, and the length does not change.
THE RELATIONSHIP BETWEEN LENGTH AND TENSION
The structural makeup of skeletal muscle creates unique properties of force production based on muscle length (Figure 1). The length of the sarcomere at the time of contraction affects the amount of force produced, because of the amount of overlap between actin and myosin molecules. The overlap is greatest at resting length; as a result, more cross-bridging occurs, and greater force is achieved. The force of contraction drops off exponentially as a muscle fiber is shortened or lengthened. These anatomic properties of skeletal muscle are significant in determining biomechanical strength, running speed, and jumping height.
THE RELATIONSHIP BETWEEN FORCE AND VELOCITY
The force-velocity relationship also is a property of skeletal muscle mechanics. As originally described by Hill in isometrically stimulated amphibian muscle, the velocity of a muscle contraction is related to the load placed on the muscle (Figure 2).1 The larger the load, the lower the velocity at which the muscle can contract. In contrast, muscle velocity increases with a light load. This relationship applies only to the initial concentric action after isometric stimulation. In eccentric contraction, the force-velocity curve is different; an eccentrically loaded muscle lengthens rather than shortens. Because muscle becomes stiffer when it resists shortening, a large change in load results in a smaller change in velocity. Eccentric contractions produce more force at the same movement velocity and therefore require less energy expenditure, which is clinically important. For example, in normal gait, the quadricep is most active eccentrically during heel strike, while the knee is flexing, and is most active concentrically during knee extension.
FIBER TYPES
Skeletal muscle is a heterogeneous tissue. The myofibers frequently are grouped based on their contraction speed and predominant energy pathways. This heterogeneity is the result of different structural and metabolic properties of the fibers. Variability in isoforms of the contractile and structural proteins can result in different fiber types. Most important are the types of myosin adenosine triphosphatase, which produce different shortening speeds (the fast and slow forms of myosin).
The three general fiber types are slow oxidative (type I), fast oxidative-glycolytic (type IIA), and fast glycolytic (type IIB). Type I fibers are smaller, contract more slowly, and produce less force than type II fibers, but are fatigue resistant. Type II fibers are larger, contract more quickly and forcefully, and are more easily fatigued. Type IIA is considered intermediate; type IIB is the fastest, most powerful, and most fatigable type. When motor units are activated by the central nervous system, the smallest motor units (predominantly type I), are recruited first; the larger type IIA and IIB units are recruited when more intense contractions are required.
The three general fiber types are slow oxidative (type I), fast oxidative-glycolytic (type IIA), and fast glycolytic (type IIB). Type I fibers are smaller, contract more slowly, and produce less force than type II fibers, but are fatigue resistant. Type II fibers are larger, contract more quickly and forcefully, and are more easily fatigued. Type IIA is considered intermediate; type IIB is the fastest, most powerful, and most fatigable type. When motor units are activated by the central nervous system, the smallest motor units (predominantly type I), are recruited first; the larger type IIA and IIB units are recruited when more intense contractions are required.
ENERGY PATHWAYS
ATP is the common energy source for muscle contraction. The intracellular stores of ATP are limited, however. ATP is regenerated to supply energy for contracting muscle through three pathways. Creatine phosphate is dephosphorylated by mitochondrial creatine kinase to convert adenosine diphosphate to ATP. Creatine phosphate reserves are adequate for short-duration (10 to 20 s), high-intensity contractions, as required for sprinting. After a maximum of 30 seconds of exercise, muscle glycogen is converted to ATP through anaerobic glycolysis, and lactate is produced. In sustained exercise of more than approximately 1 minute, aerobic oxidation of glucose and fatty acids predominates, allowing a much more efficient energy yield. While anaerobic oxidation yields 2 ATP per molecule of glucose, aerobic oxidation yields 32 per molecule. Cardiac output and total ventilation increases dramatically to provide the oxygen required to support aerobic oxidation. The endurance limit (approximately 370 W in elite athletes) is the maximum sustainable energy output, as restricted by the rate of oxygen delivery and aerobic oxidation. Beyond this limit, anaerobic glycolysis can temporarily compensate through the rapid production of lactate. Increasing lactate and H+ ion production causes a systemic drop in pH, inhibiting the reactions that drive muscular contraction. At a plasma lactate level of approximately 4 mmol/L, the anaerobic threshold is reached; at this point, performance declines, and rapid fatigue ensues.
MUSCLE GROWTH AND ADAPTATION
DEVELOPMENT
Skeletal muscle arises from mesodermal somite tissue. Early muscle progenitor cells are called myoblasts, and the fusiform cells that eventually fuse into multinucleated cells are called myotubes. Many contractile elements appear in the cell at the approximate time of myotube formation. Further differentiation occurs with muscle-specific expression of contractile proteins, elements of the metabolic pathways, and connections to the extracellular matrix.
During normal development, skeletal muscle grows in volume and length. To accommodate skeletal growth, total muscle length increases but sarcomere length remains constant. Additional sarcomeres are added in series near the region of the myotendinous junction. This mechanism persists at skeletal maturity, when muscles immobilized under stretch increase in length. Initially, the myofibrils and sarcomeres of a stretched muscle are lengthened. Over a period of weeks, additional sarcomeres are added so that resting sarcomere length is restored, but the entire muscle remains at the new, stretched length. The mechanical properties of a lengthened muscle also change; the length-tension curve shifts to produce peak tension at a greater length and to produce less passive force when stretched. Conversely, greater passive force is generated by the same stretch if a muscle is shortened for a prolonged period of time. Clinically, the mutability of muscle length has been advantageously applied in surgical limb-lengthening and muscle transfer procedures.
IMMOBILIZATION
Muscle undergoes several changes in response to immobilization or disuse. When skeletal muscle is immobilized, atrophy quickly ensues because of many factors including reduced protein synthesis and hormonal contributions. The result is a loss of cross-sectional area, leading to decreased muscle strength. Fatigability increases, partly because of diminished energy stores and metabolic efficiency. The response to immobilization depends on muscle length, however. Atrophy, loss of extensibility, and loss of force production are more pronounced in muscle immobilized without tension than in muscle immobilized under stretch. When muscles are stretched in immobilization, the loss of strength is partially compensated for by the growth in length, with formation of new sarcomeres and contractile proteins. Stretched muscle also produces less tension from stretch and maintains its extensibility. Although prolonged immobilization should be avoided, attention to joint position and muscle length can optimize future function.
ATROPHY
Skeletal muscle atrophies during periods of decreased muscle stimulation or systemic illness, as during immobilization or bed rest (eg, in burn treatment or surgery). The mechanisms driving disuse atrophy are a decrease in muscle protein synthesis and an increase in muscle protein breakdown.2 As the body ages, skeletal muscle atrophies and muscle function gradually declines with decreased force production, contraction velocity, and impaired relaxation.3 Sarcopenia (degenerative loss of skeletal muscle) was found in approximately 25% of people aged 65 to 70, and 40% of those older than 80 years.4 Resistance exercise training and nutritional supplementation have been shown to improve the results of rehabilitation and to reduce muscle wasting in disuse, aging, or disease states.5
TRAINING
Under the demands of physical training, the skeletal musculature undergoes a range of adaptations to improve muscle performance. The types of training and their primary effects can be simplified into three categories: motor learning, endurance training, and resistance training. Motor learning to improve the accuracy and performance of motor skills primarily results in nervous system adaptations such as the timing and rate of contractions. Endurance training (eg, in distance running) focuses on increasing the oxidative capacity of slow-twitch (type I) fibers, mainly by increasing mitochondrial density and cardiac output. Resistance training (eg, in weight lifting) uses relatively short, powerful contractions and requires training the fast-twitch (type II) fibers. Adaptations result from increases in the cross-sectional area of the muscle as well as improved neural activation.
MUSCLE INJURY
Muscle injury commonly occurs during physical activities and in almost all patients with skeletal trauma. Muscle injury usually is found with a strain, contusion, or laceration. Regardless of the mechanism of injury, the pattern of biologic response is muscle degeneration and inflammation followed by regeneration and fibrous scar formation (Figure 3).
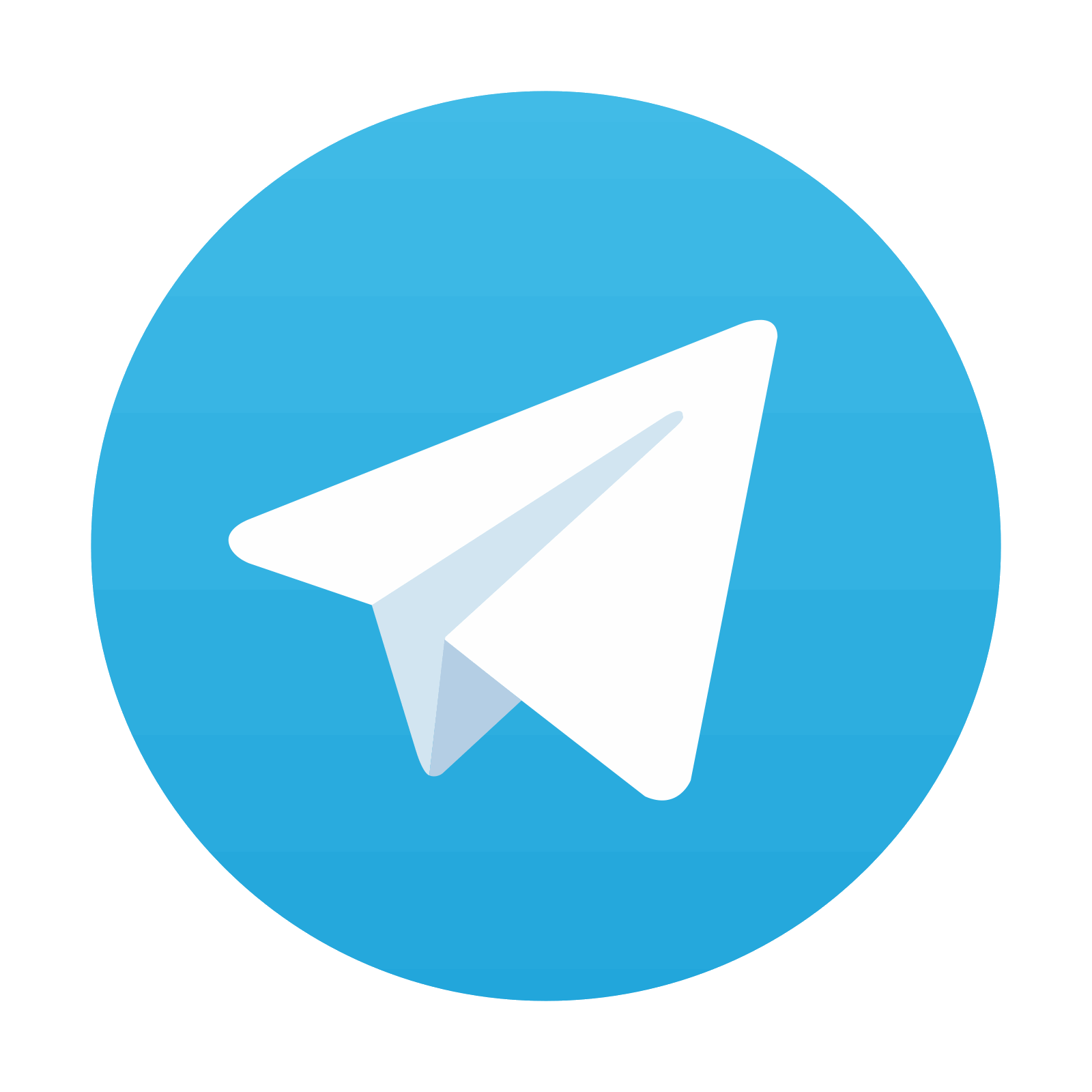
Stay updated, free articles. Join our Telegram channel
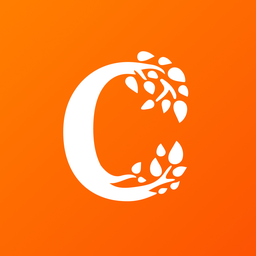
Full access? Get Clinical Tree
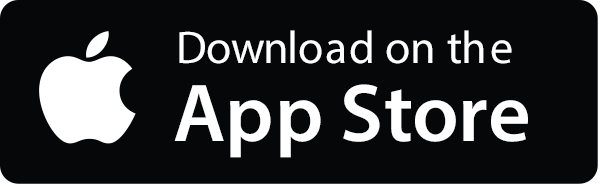
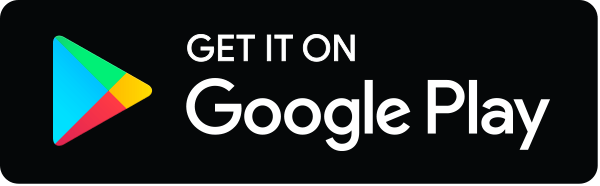