Fixation by Ingrowth
Vikram Chatrath
Murali Jasty
Paul E. Beaulé
Heino Kienapfel
Peter Griss
Historical Background
The first patent for the concept of fixation by bone ingrowth was issued to Greenfield (1) in December 1909. Introducing a metallic cagelike framework for an artificial tooth root, Greenfield suggested that in the course of time the bone would grow in, around, and through the frame, and the latter would therefore be held securely in position. However, this concept did not gain wide acceptance for many years. In the 1950s, the use of porous polyvinyl sponges (2,3) was investigated for the reconstruction of bone. Struthers (4) proposed their use as augmentation for autogenous grafting procedures. Other investigators (5,6,7) focused on a resorbable porous polyurethane and Teflon for osteosynthesis.
The first porous material that appeared to have adequate mechanical strength for a load-bearing orthopedic application was Cerosium, a porous ceramic–plastic composite introduced by Smith (8) in 1963. He postulated that porous materials such as these would be biologically and biomechanically compatible with bone. However, the pore sizes in the material (the pores were to allow the ingrowth of bone into the material for mechanical attachment of the implants) were too small (1 to 25 μm) to allow the ingrowth of bone.
The late 1960s and the early 1970s were particularly fruitful for the evolution of porous materials for prosthetic coatings. Hulbert (9) recognized the importance of pore size and material strength for bone ingrowth. He advocated porous ceramics to allow bone ingrowth and provide mechanical anchorage for prosthetic implants. In 1968, Hirschhorn and Reynolds (10) were the first to report on the fabrication of porous metal (cobalt-chromium alloy) as implant materials in 1968, using powder metallurgical techniques that became available at the time. In 1969, Lueck et al. (11) reported the fabrication and implantation of a porous, commercially pure titanium fiber composite material. They suggested that porous metallic materials produced by powder metallurgy techniques exhibit poor strength characteristics when the degree of porosity is sufficient to permit bone ingrowth. Ever since, the fiber–metal material pioneered by Rostoker and Galante (12) has been under continuous investigation (13), and it is currently used clinically as a coating on total hip prostheses (14,15) and total knee prostheses (15). In Europe, Ducheyne et al. (16,17) developed stainless steel porous coatings with pore sizes ranging from 50 to 100 μm and performed animal experimental studies under dynamic loading conditions.
In the 1970s, research studies on porous cobalt-chromium were continued by Welsh, Pilliar, and Cameron (18,19,20,21,22,23) and developed further by Bobyn (24,25,26) and others. They prepared specimens by sintering microspheres of the alloy either greater or less than 44 μm in diameter. Based on mechanical push-out tests in a canine cortical bone model, they concluded that both the small and large pore-size materials were effective. This research was the basis for the porous cobalt-chromium alloy coatings currently in use (27,28).
Porous polymers, such as porous polyethylene (29) and porous polysulfone (30) have also been investigated but have not received widespread use because of wear and inadequate mechanical strength. A composite of Teflon–graphite fiber (Proplast) material (31) was used clinically for a period of time, but eventually discontinued because of high failure rates.
Current metallurgical techniques allow the fabrication of a wide variety of porous-surfaced implants with a variety of materials, pore sizes, pore geometries, and attachments of porous coatings to solid metal substrates of the implants (Fig. 46.1). The majority of total joint prosthesis used today employs the biologic fixation methods using porous-surface metal implants. The subsequent sections in this chapter discuss requirements and the results in the clinical use of these implants as load-bearing members in total joint replacements.
Basic Requirements for Ingrowth
The principal requirements for osseointegration (32,33) and bone ingrowth (34,35) are now well understood. The term osseointegration refers primarily to the attachment of implants to bone where the implants become part of the bone by (a) ingrowth into the porous surfaces or by (b) ongrowth onto the surface of an implant that is not porous but rough or textured on a microscopic scale (32,36). The term bone ingrowth refers to actual bone formation within the porous-surface structure of an implant and the interconnection of ingrown bone spicules within the porous coating. This chapter primarily discusses the latter phenomenon, although
there is evidence from animal experimental studies that a textured surface (i.e., a surface roughening by grit blasting) also is excellent for bone–implant attachment (37,38). Thus bone ingrowth in this context refers to interconnecting bone within the porous coating that is a three-dimensionally interconnecting porous layer.
there is evidence from animal experimental studies that a textured surface (i.e., a surface roughening by grit blasting) also is excellent for bone–implant attachment (37,38). Thus bone ingrowth in this context refers to interconnecting bone within the porous coating that is a three-dimensionally interconnecting porous layer.
![]() Figure 46.1. Cross section through some common porous coatings. Top: Plasma-sprayed coating. Middle: Sintered sphere coating. Bottom: Fiber mesh coating. |
The physiologic response to a porous-coated implant inserted into bone after rasping or reaming the bone resembles the healing cascade of cancellous defects, with the newly formed tissues occupying the void spaces of the porous material. Repair of a hole drilled within the medullary canal of a long bone or a cavity created by reaming the subchondral bone of the acetabulum is associated with the formation of a hematoma and development of mesenchymal tissue, which is replaced by woven bone. Intramembranous bone formation is seen in and around the porous coating in as little as a week after surgery, and extensive woven bone is seen in the porous coating by 3 weeks. Lamellar bone remodeling soon follows, as does reestablishment of the bone marrow usually by around 6 weeks. As in primary fracture healing with stable osteosynthesis (39), no intermediate fibrocartilaginous stage occurs. However, rare areas of callus and endochondral bone formation can be seen with porous-coated implants, probably reflecting the small amount of micromovements that occur at the bone–porous coating interface. Consequently, the clinical success of biologic fixation by bone ingrowth depends on a stable implant–bone interface. Implants that obtain only fibrous tissue ingrowth rather than bone ingrowth can function well on occasion but not as reliably and consistently as those that obtain bone ingrowth.
Biocompatibility of Implant Materials
Various metallic, ceramic, and polymeric implant materials have been introduced in joint replacements over the years. These implant materials are known to demonstrate different patterns of biocompatibility. Based on animal experimental studies, the reactive new bone formation adjacent to the material surfaces can be categorized (40), as distance osteogenesis, contact osteogenesis, and bond osteogenesis (Table 46.1). However, transmission electron microscopy studies have demonstrated that the implant–bone ultrastructures for chemically pure (CP) titanium (Ti), Ti6Al4V, cobalt-chromium (CoCr) alloy, and stainless steel were comparable.
Accordingly, various porous-surface technologies have been developed, including porous metals (titanium (11,12)), cobalt-chromium-molybdenum alloy (10,23,36), and stainless steel (17,41), porous polymers (Teflon, polyethylene, polysulfone, and polypropylene (29,30,42,43,44)), porous carbon (43,45), and porous ceramics (9,42,44). Only the porous coatings of titanium, cobalt-chromium-molybdenum, Teflon, polysulfone, and polyethylene have been used clinically. Presently, the porous materials that are most commonly used are porous metal coatings because of their excellent biocompatibility and strength. These coatings are applied by sintering techniques (CoCr microspheres, CoCr fiber–metal composites, CP Ti fiber–metal composites, and CP Ti microspheres). One of the sintering techniques is diffusion bonding, where metal titanium fibers are pressed to a solid titanium substrate under pressure and temperature so as to weld the fibrous porous coatings onto the metal substrate. More recently, trabecular metal (TM) surfaces made
of tantalum materials, which have greater porosity than sphere or fiber coatings, have also become popular.
of tantalum materials, which have greater porosity than sphere or fiber coatings, have also become popular.
Table 46.1 Biocompatible Materials and Their Reactive Bone Formation | ||||||||||||||||||||||||||||||
---|---|---|---|---|---|---|---|---|---|---|---|---|---|---|---|---|---|---|---|---|---|---|---|---|---|---|---|---|---|---|
|
Experimental Models for the Assessment of Bone Ingrowth
Numerous animal models have been used to assess bone ingrowth. Models using rodents appear to have the disadvantage of a comparatively high bone formation rate. The bone formation rates of primates, canines, sheep, and pigs are closer to human bone formation rates. Therefore, the majority of experimental studies were done using the later models and employed quantitative means to assess bone ingrowth and strength of fixation (46,47,48,49,50). A recent study comparing primate and canine models for bone ingrowth experimentation found no significant difference in bone growth into the experimental plugs between the two animal models, indicating the acceptability of cross-species interpretation of existing data from dogs (51).
Most models were used for both weight-bearing and non–weight-bearing study designs. In both types of models, either the implants are placed in a press-fit fashion to ensure intimate contact with the host bone or interfacial gaps are created by overdrilling or over reaming. In the press-fit model, the control is usually an untreated press-fit implant. In the gap studies, a negative control is provided by an untreated gap, and a positive control is provided by a press-fit implant.
A more complicated though clinically more relevant approach is to use a weight-bearing revision model. In this model, an initial implant is fixed by bone cement in a manner predisposing to loosening of the implant. The loosening process is accompanied by and presumably caused by a histiocytic response and bone resorption. Once the cemented implant is loose, it is surgically removed, and the failed prosthesis is replaced with a cementless porous-coated revision implant. Because of the loosening process, the medullary cavity in the region of the implant is devoid of normal marrow, and gaps are present between the revision implant and the host bone. In the revision studies, a negative control is provided by untreated implants. The negative control is needed to determine if the graft material has an enhancing effect (i.e., to determine if it improves the amount of bone ingrowth or the strength of fixation of the implant). A positive control is useful in that it indicates the maximal amount of bone ingrowth or fixation strength that can reasonably be expected.
Pore Size and Shape and Porosity
In order for bone ingrowth into the porous coating to occur, appropriate pore size must be provided. Bone trabeculae and osteons are on the order of tens of micrometers thick, and pore size should be at least on this order; otherwise bone ingrowth would not occur. A pore size of greater than 100 μm would allow structural organization to the ingrown bone (trabecular and osteonal spaces) and would be desirable. However, a pore size greater than 500 μm up to a millimeter would resemble a macrotextured surface rather than a three-dimensionally interconnecting porous surface and theoretically would lead to loss of interface shear strength. The effect of pore size on the strength of fixation has been investigated in canine models using pore sizes ranging from less than 50 μm to 800 μm. In studies examining pore sizes less than 100 μm, increased pore size was associated with increased strength of fixation (23,52). Most studies analyzing pore sizes in the range 150 to 400 μm have shown no relationship between pore size and strength of fixation (13,26,53). In a weight-bearing canine total hip replacement model, it has been reported that more bone ingrowth was observed in acetabular components with 450- and 200-μm pores than in those with 140-μm pores (48). From these studies, it can be concluded that the optimal range for pore sizes is 100 to 400 μm. Most of the porous-coated prostheses currently in use have pore sizes in this range (54).
The porosity of the porous-coating layer is also an important parameter governing the fixation of porous-coated implants. Sintering techniques in current clinical use provide porosities of around 30% to 50%. The wire mesh and cancellous structured porous coatings generally provide greater degrees of porosity without compromising the structural integrity of the porous coatings than do the plasma-sprayed or sintered sphere porous coatings. Some of the early implants with plasma-sprayed and sintered sphere coatings had problems with the coating separations, but most coatings used today have adequate strength and porosity to be successful in clinical use.
Another important parameter determining the mechanics at the bone–porous coating interface is the interconnecting pore size. This is the size of the pore where one pore interconnects to another pore within the porous coating. Larger numbers of interconnecting pores with adequate interconnecting pore sizes lead to better strength at the bone–porous coating interface, particularly in tension, owing to the three-dimensional interconnectivity of the ingrown bone. The interconnecting pore sizes are partly dependent on the techniques used to fabricate the porous surface. In general, the connecting pores are larger in fiber–metal and TM porous coatings and wire mesh porous coatings than the connecting pores in sintered porous coatings, which in turn are larger than the connecting pores in plasma-sprayed or corundum roughened surfaces. This is because the porous-surface geometry can be better controlled with the meshes than it can be with spheres or sprayed coatings. For example, the pore geometry and size are determined by the diameter of the spheres and their spacing in sintered sphere coatings. Excessively increasing the size of the spheres or packing them too loosely to increase the interconnecting pore sizes would lead to loss of the interconnections (or welds) between the spheres and thus to loss of porous coating strength. Current sintering techniques, however, are able to provide adequate strength of porous coatings and of the bone–porous coating interface to maintain firm and long-lasting fixation of implants to the skeleton with any of the porous coatings in clinical use. Some of the newer porous coatings, such as the TM porous coating, are thought to have the advantage of providing increased porosity and interconnecting pore sizes while retaining high strength of the porous coating (Fig. 46.2).
Implant Stability or Micromotion
Uncemented porous-coated implants require bone ingrowth for long-term stability. Rigid initial fixation by mechanical means is necessary for these implants to allow bone ingrowth that provides long-term stability. The initial fixation is provided by a variety of surgical techniques, such as ancillary fixation with screws, ancillary fixation with projections from the implants (e.g., spikes and pegs), or press-fit fixation where the implant is driven into a cavity that is slightly undersized compared with the dimensions of the implant. The particular method of initial fixation employed depends on the types of implants and the location of their use as well as the philosophy of the designers. Most acetabular components are fixed by press fitting a hemispherical component into a slightly undersized hemispherical cavity and by supplemental fixation employing screws inserted through the component into the adjacent bone. Most femoral components are fixed by press fitting a cylindrical or conical implant into the femoral canal, which is prepared to be slightly smaller than the implant either in the diaphysis or the metaphysis.
The implants that are designed to have bone grow into the porous surface rely on a tight initial fit within the bone cavity. Over time, bone is expected to grow into the interconnected pores and firmly incorporate and fix the implant to the bone. If the bone grows into and remains in the pores, these implants should theoretically provide a stable and permanent fixation. Unfortunately, even the very slightest motion between the implant and bone may inhibit, prevent, or delay the growth of bone into the pores. There is evidence that too much relative motion between the implant and host bone leads to ingrowth of fibrous connective tissue rather than bone. The extent of implant stability contributes to a reduction in relative motion between the implant and host bone (55,56,57,58).
It has been demonstrated in experimental studies in which fixed micromotions were applied to porous-coated implants in vivo that micromotions of 150 μm induce fibrous tissue ingrowth (46,59). Micromotions of 150 μm did allow the formation of woven bone within a porous titanium wire surface (Fig. 46.3). However, there appeared to be no osseous continuity between the woven bone within the coating and the trabecular bone adjacent to the implant. The interface formed under displacements of 40 μm was composed of a mixture of bone and fibrous tissue. The interface formed under displacements of 0 μm and 20 μm consisted predominantly of bone ingrowth without intervening fibrous tissue or fibrocartilage.
![]() Figure 46.3. Fibrous tissue formation at the interface of a porous-coated plug that had induced motion of 150 μm in vivo. |
Although the studies just described employed fixed displacements to implant plugs in bone, other studies measured the initial stability of femoral components in canine femurs and compared that to the femoral component stability in vivo after bone ingrowth had taken place (60). These studies showed that an average initial micromotion of around 60 μm was found immediately after femoral component insertion, but excellent bone ingrowth occurred with this degree of motion. The bone ingrowth markedly increased the stability of the femoral component, which measured less than 10 μm after bone ingrowth had taken place.
Recent roentgen stereophotogrammetric analysis (RSA) in vivo studies in porous-coated THA and total knee arthroplasty (TKA) components have demonstrated that in many cases the analyzed micromotions were well above the limits of bone ingrowth compatibility (61,62,63,64,65). Bone ingrowth can occur even with such movements.
Additional hydroxyapatite (HA) coating demonstrated an effect on the architecture of fibrous tissue formation in a weight-bearing canine gap model, allowing controlled micromovements of 150 μm. In this study (66), both the HA-coated and the titanium porous–coated implants had only fibrous tissue ingrowth. However, there was a stronger fibrous anchorage of the HA-coated implants, together with the presence of fibrocartilage, higher collagen concentration, and radiating orientation of the collagen fibers.
It is difficult to define accurately the initial stability required for bone ingrowth in terms of micromotion, however. This is because the implants are subjected to fixed loads and not fixed displacements in vivo. During the days and weeks following implantation, an implant is gradually further stabilized by endochondral or intramembranous bone formation, maturation of the ingrown bone, and remodeling, each of which reduces the micromotion under a given load. Although all of this is going on, the amount of initial press fit decreases, due to relaxation of surrounding bone
and its remodeling. Thus it is difficult to determine accurately the initial micromotion below which bone ingrowth occurs and above which fibrous tissue ingrowth occurs.). It is generally assumed, however, that initial stability of the prosthetic component with micromotions less than about 100 μm should be maintained for bone ingrowth to occur.
and its remodeling. Thus it is difficult to determine accurately the initial micromotion below which bone ingrowth occurs and above which fibrous tissue ingrowth occurs.). It is generally assumed, however, that initial stability of the prosthetic component with micromotions less than about 100 μm should be maintained for bone ingrowth to occur.
It can be concluded that bone ingrowth depends on an optimal primary stability. From a clinical perspective, this primary stability can vary depending on implant design variables (cross-sectional geometry, means of additional fixation, mismatch in implant–bone stiffness), implantation technology variables (accuracy of tools for rasping, reaming, drilling, sawing), surgical technique variables (accuracy of utilization of the implantation technology), and patient variables (bone quality, bone defects). Most of the contemporary implants and current surgical techniques are adequate to achieve bone ingrowth fixation of porous-surfaced implants consistently and reliably.
Interface Distances
The lack of a direct and continuous interface contact between the porous surface and the host bone has a negative effect on bone ingrowth and strength of fixation in both weight-bearing and non–weight-bearing models. Numerous gap models with a controlled gap and a stable implant have demonstrated the inhibiting effect of gap size on bone ingrowth and strength of fixation.
Laboratory animal studies employing canine total hip models have shown that in weight-bearing porous-coated acetabular components, gaps between the bone and porous coatings as little as 0.5 mm at the time of implantation adversely affect the bone ingrowth and lead to fibrous tissue formation (48). Current surgical techniques, instruments, and implants allow this degree of precision in primary surgery, though some gaps are unavoidable in revision surgery.
A 2-mm non–weight-bearing gap model with a Ti porous-coated implant was used to analyze the effect of an additional HA coating. After an in situ duration of 3 weeks, the amount of bone ingrowth was six times higher for the press-fit–inserted implants than for the contralateral 2-mm gap control (54,67
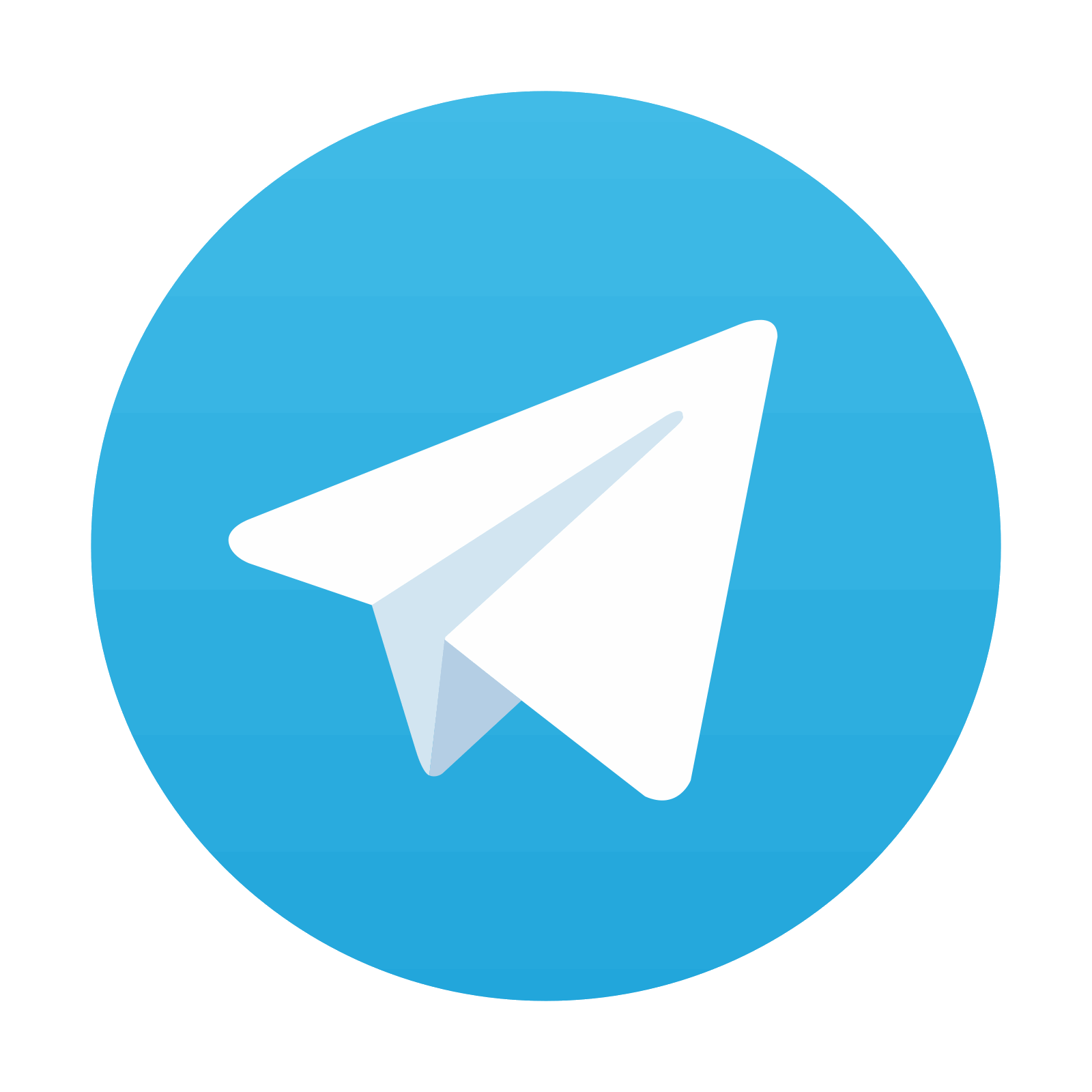
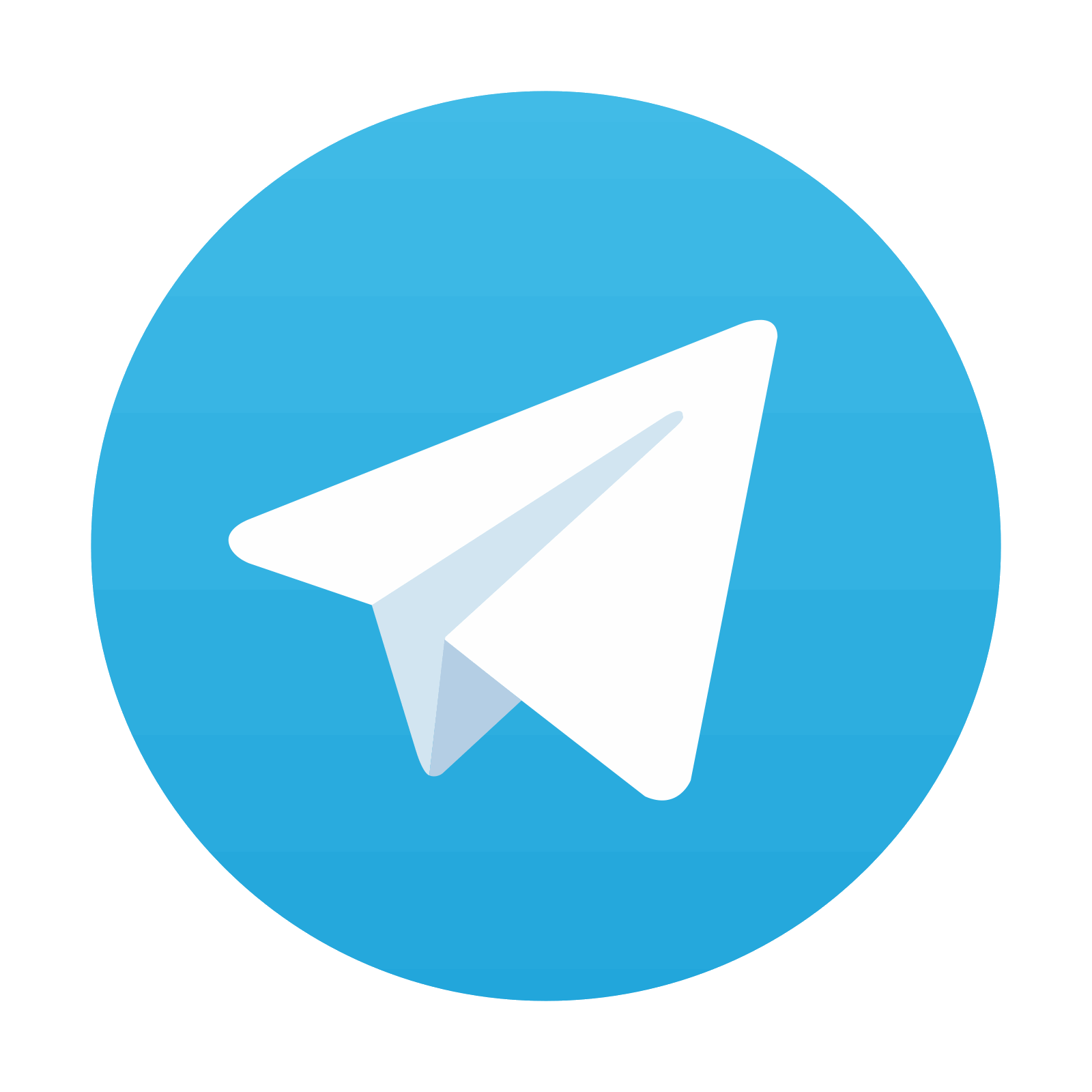
Stay updated, free articles. Join our Telegram channel
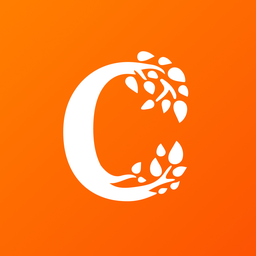
Full access? Get Clinical Tree
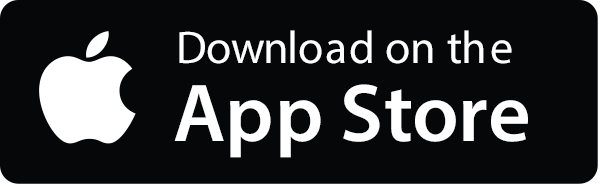
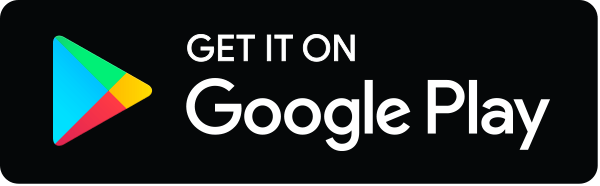