Bandwidth name
Frequency (Hz)
Delta
0–4
Theta
4–8
Alpha
8–13
Beta1
13–24
Beta2
24–32
Gamma
32–60
Alpha activity can be seen in about three-fourths of all individuals when they are awake and relaxed. Asking these individuals to further relax and close their eyes will result in recurring periods of several seconds in which the EEG consists of relatively large, rhythmic waves of about 8–12 Hz. This is the alpha rhythm, the presence of which has been related to relaxation and the lack of active cognitive processes. If someone who displays alpha activity is asked to perform cognitive activity such as solving an arithmetic problem in his or her head, alpha activity will no longer be present in the EEG. This is referred to as alpha blocking. Typically, cognitive activity causes the alpha rhythm to be replaced by high frequency, low amplitude EEG activity referred to as beta activity. Since the discovery of the alpha rhythm, a variety of studies have focused on its relationship to psychological processes and broad developments of the cognitive and affective neurosciences amplified this interest (see [25, 26] for reviews).
High frequency activity occurs when one is alert. Traditionally, lower-voltage variations ranging from about 18 to 30 Hz have been referred to as beta and higher frequency lower-voltage variations ranging from about 30 to 70 Hz or higher are referred to as gamma. Initial work suggests that gamma activity is related to the brain’s ability to integrate a variety of stimuli into a coherent whole. For example, Catherine Tallon-Baudry and her colleagues [27] showed individuals pictures of a hidden Dalmatian dog that was difficult to see because of the black and white background. After training individuals to see the dog, differences in the gamma band suggested meaningful and non-meaningful stimuli produced differential responses.
Additional patterns of spontaneous EEG activity include delta activity (0.5–4 Hz), theta activity (5–7 Hz), and lambda and K-complex waves and sleep spindles, which are not defined solely in terms of frequency. Theta activity refers to EEG activity in the 4–8 Hz range. Grey Walter [28], who introduced the term theta rhythm, suggested that theta was seen at the cessation of a pleasurable activity. More recent research associated theta with such processes as hypnagogic imagery, REM (rapid eye movement) sleep, problem solving, attention, and hypnosis. Source analysis of midline theta suggests that the anterior cingulate is involved in its generation [29]. In an early review of theta activity, Schacter [30] suggested that there are actually two different types of theta activity: First, there is theta activity associated with low levels of alertness as would be seen as one falls asleep. Secondly, there is theta activity associated with attention and active and efficient processing of cognitive and perceptual tasks. This is consistent with the suggestion of Vogel et al. [31] that there are two types of behavioral inhibition, one associated with a gross inactivation of an entire excitatory process resulting in less active behavioral states and one associated with selective inactivity as seen in overlearned processes.
Delta activity is low frequency (0.5–4 Hz) and has been traditionally associated with sleep in healthy humans as well as pathological conditions including cerebral infarct, contusion, local infection, tumor, epileptic foci, and subdural hematoma. The idea is that these types of disorders influence the neural tissue that in turn creates abnormal neural activity in the delta range by cutting off these tissues from major input sources. Although these observations were first seen with intracranial electrodes, more recent work has found similar result using MEG and EEG techniques. Additionally, EEG delta activity is the predominant frequency of human infants during the first 2 years of life.
EEG and Concussion
While conventional EEGs are not part of the current clinical “gold-standard” assessment battery, a number of studies show EEG differences in those individuals suffering from mTBI compared to healthy controls (see [32] for an overview). Of the differences observed on conventional EEGs, the most common abnormalities seen are generalized or focal slowing as well as weakened posterior alpha in mTBI patients [14, 33, 34]. These deficits were found in the immediate post-injury period (within a few hours of a concussive episode); however, similar findings have been reported even when there is a longer period between injury occurrence and injury evaluation.
These common abnormalities seen on conventional EEG recordings usually resolve within the first few months post-injury [35], similar to the resolution of functional and symptomatic deficits in typical concussive recovery. However, up to 10 % of individuals diagnosed with mTBI still show atypical electrophysiological readings in the late post-injury period [35, 36]. This small but significant portion of individuals who show electrophysiological abnormalities in the late post-injury period parallels those individuals who have atypical resolution of concussive symptoms and functioning.
Traditionally, in clinical settings, conventional EEGs were interpreted by the visual inspection of raw EEG signals. However, studies show that visual inspection of EEG lacks the sensitivity to detect changes following mTBI. With the advancement of computerized signal processing techniques, there is a growing body of literature that suggests more complex EEG paradigms may be used to assess changes in functional status after concussive injuries [3]. Compared to visually inspected EEGs, computerized EEG analyses are advantageous because they can detect subtle differences in signal patterns and shifts not visible to the naked eye [8]. Due to these benefits, Cannon et al. [37] indicated that the usefulness of EEG as an assessment tool for brain injury is due to its “direct signature of neural activity” and “ideal temporal resolution.”
Several different types of variables can be isolated using quantitative EEG methods. Spectral analysis, relative amplitude and power in a particular frequency bandwidth, coherence, and phase are the most common types of analyses performed in EEGs. In terms of TBI, frequency and coherence analyses of particular cortical areas can offer important information [3, 8, 38]. By examining the pattern of activity between the cortical areas, it is also possible to delineate brain networks, see how they are involved in different types of tasks, and determine how they differ under certain conditions such as the presence of a concussion.
Coherence analysis describes how the EEG signal at each of two electrodes is related to one another. In simple terms, coherence reflects the manner in which two signals “covary” at a particular frequency. In specific, coherence measurements represent the correlation of signal phase stability between two different electrodes. Coherence measures within the same frequency band offer an estimate of the temporal relationships between adjacent neural systems. Like correlation, coherence is a measure between 0 and 1, where 1 represents a perfect phase correlation between two groups and 0 represents no correlation. Thus, in performing the coherence analysis, one can also obtain a measure of phase or synchrony.
The particular interest in EEG coherence is due to the biological nature of concussive injury. The brain structures involved in neural connectivity, such as the reticular system activation and thalamocortical tracts, are the structures most likely to be affected by mTBI. Considering the probability that these areas are altered following concussion, frequency and coherence analyses are likely to be the most sensitive electrophysiological measures to indicate deficits due to concussive injury.
According to Arciniegas [8], frequency measures can vary with the number of neurons (smaller number, smaller amplitude/power), the integrity of the thalamocortical circuits in which the neurons contribute (injury to the circuit causes slower frequencies), and the influence of activation from the reticular system (increases in reticular system activity cause higher frequencies, while decreases in reticular system activity cause lower frequencies).
Coherence, which by definition correlates the frequency measures between two different electrodes, may indicate the level of communication between different areas of the brain and signify neural network connectivity and dynamics [8]. Reduced coherence values can be attributed to damage in myelinated fibers and/or gray matter [38]. If lowered coherence values are seen in mTBI patients, it is still unknown which of these factors, or if a combination of all of them, produce these results.
Each concussive episode is individualized and may produce different changes in the brain. In turn, one might expect that the respective EEG measures would be different in each mTBI patient. While the electrophysiological deficits found for each concussive episode remain unique, several consistent EEG patterns have been identified. According to a review by Arciniegas [8], the most common EEG findings in mTBI include: (1) a decrease in mean alpha frequency [16, 19, 39–42], increase in theta activity [15, 17, 43, 44], or increased alpha–theta ratio [18, 19, 39, 42] and (2) lessened alpha and beta power between anterior and posterior regions, weakened alpha power (posterior region), and increased coherence between frontal and temporal regions [45–47].
Along with these findings, a literature review by Nuwer et al. [34] listed other common EEG findings after concussive episodes. These findings concluded that changes in EEG measures resolved along the same timeline as symptoms, with gradual changes mainly occurring over weeks to months. The researchers also found that left temporal slowing may correspond to lingering cognitive symptoms. In all the studies evaluated by Nuwer, coherence was not correlated to outcome or diffuse axonal injury. Due to how quickly EEG patterns can change in an mTBI population [8], it is critically important that research involving individuals being tested after a concussive injury are evaluated in as similar time points as possible.
Evidence provided by Thornton [48] and Thatcher et al. [49] indicates that the EEG patterns seen in a concussed athlete do not quickly change over time and, therefore, should be present at the initial time of injury. While this is useful in describing EEG as a possible tool in diagnosing and evaluating concussed individuals, it also indicates that concussive episodes, even “mild” or “typical” episodes, cause long lasting alterations in brain electrophysiology. Work by Barr et al. [50] showed that despite improvement or normal levels of cognitive functioning, brain patterns remain altered in mTBI patients. This further suggests that the brain may not completely heal from concussive episodes; instead the individual learns to compensate for the deficit in order to achieve normal performance. The idea of compensation instead of recovery has been examined in a study by Thornton [51] and discussed in a book chapter [38].
Two prominent studies have examined the reproducibility of EEG absolute measures. First, a study by Corsi-Cabrera et al. [52] tested nine subjects 11 times over a 1-month period. When looking at absolute amplitude, the median correlation coefficient over the 11 sessions was 0.94. Alpha and beta bands showed greater variability than any of the other bands. Pollock et al. [53] evaluated test–retest reliability in each bandwidth over a 20-week period on 46 normal controls. Absolute amplitude in theta, alpha, and beta1 had correlation coefficients that exceeded 0.60. Beta2 and delta correlation coefficients were found to be lower, with delta showing the poorest correlation. The authors also found that absolute amplitude has higher correlation coefficients than relative power and is, therefore, recommended for use in future studies. The high levels of correlation found in these studies, combined with the varying intervals between testing sessions (a common feature in concussion testing), imply that absolute amplitude is an appropriate measure for research purposes.
Although related to amplitude, several studies have separately analyzed the reproducibility of power. Salinsky et al. [54] tested absolute and relative power and found correlation coefficients of 0.84. Tests were run between 12 and 16 weeks apart on 25 normal controls. Cannon et al. [37] examined test–retest EEG power reliability by examining 19 normative controls over a 30-day testing period. Each participant was recorded for a 4-min interval under an eyes open and eyes closed condition. Intraclass correlation coefficients (ICCs) for absolute power were 0.90 for eyes closed data and 0.77 for eyes open data. The results of these studies closely mimic those found when evaluating amplitude, with power having sufficiently high levels of reliability over both short (days) and long (months) testing periods.
Mathematically distinct from amplitude and power, researchers have spent time considering the reproducibility of coherence values. Studies by Harmony et al. [55] and Nikulin and Brismar [56] evaluated the reproducibility of coherence during rest and cognitive tasks in individuals. Both studies found good correlations within a given task or under resting state conditions but Harmony et al. reported much lower correlation values between sessions, even within the same subject during the same condition.
While these early tests show low levels of reproducibility, even within testing sessions, more recent research has provided vastly different results. The Cannon et al. [37] study mentioned earlier also examined coherence over a 30-day testing period. For eyes closed coherence measures, ICCs for delta, theta, alpha, and beta bandwidths were all greater than 0.90. For the eyes open condition, coherence in all bandwidths had ICCs above 0.85. This indicates “good” to “very good” reproducibility for all EEG variables examined and deems coherence as a reliable enough measure to use in both a research and clinical setting.
In all of the studies presented, roughly half of the variance seen in all EEG variables was reproducible within the given subject. These measures have all been determined to have a sufficient level of reproducibility to use in future research. However, it should be noted that these results do not necessarily indicate that EEG can currently be considered a reliable diagnostic tool and differentiate between concussed and healthy individuals.
Although there are many benefits to using EEGs in concussion research and a wealth of knowledge has been gained, the use of EEG in this type of research is not without its criticisms and limitations. Nuwer et al. [34] have questioned the use of EEG in concussion research, citing the lack of clear EEG features that are specifically unique to mTBI patients, especially late after injury. While there is merit to a lack of unique abnormalities, several studies [57, 58] have found deficits in concussed participants up to 3 years post-injury.
Most EEG and concussion research focus on lower frequency bands, but several studies by Thornton [38, 48, 51, 58] demonstrated that extending the frequency to include gamma bands provides important additional information, particularly between correlating EEG variables and the participant’s cognitive deficits. Additionally, most research and, consequently, normative databases provide information solely about eyes closed conditions. This severely limits the type of cognitive testing that can be simultaneously completed; restricting neuropsychological testing to auditory-based tests. While auditory-based cognitive research has provided valuable EEG patterns, such as those outlined in Thorton and Carmody [38], several cognitive domains cannot be adequately assessed via auditory tasks. The link between EEG patterns and cognitive domains, such as visual memory and attention, remains poorly established and under-researched.
In summary, reviews by Arciniegas [8] and Nuwer et al. [34] have cited numerous studies that have proven EEG as a useful tool for identifying and managing concussive injuries. While EEGs are one of the least expensive and easiest to use neuroimaging tools, the expertise needed to administer and evaluate EEG results, as well as the lack of research between EEG and concussion, has kept EEG evaluations from becoming part of the current clinical gold standard. The most comprehensive EEG study using a database of 608 mTBI subjects that were followed up to 8 years post-injury revealed a number of findings. These include: (a) increased coherence in frontal–temporal regions; (b) decreased power differences between anterior and posterior cortical regions; and (c) reduced alpha power in the posterior cortical region, which was attributed to mechanical head injury [47]. A study by Thornton [58] has shown a similar data trend in addition to demonstrating the attenuation of EEG within the high frequency gamma cluster (32–64 Hz) in mTBI patients. Overall, resting EEG has demonstrated alterations in power dynamics across electrical spectra [8], increased short distance coherences [59], and decrease in connectivity across long-distance connections [59]. These consistent findings in resting EEG and mTBI research point to the sensitivity and validity of using EEG in the assessment and management of concussion. However, it should be noted that one controversial report concluded that no clear EEG features are unique to mTBI, especially late after injury [34].
Current Work from Our Lab
In our work, significant reduction of the cortical potentials amplitude and concomitant alteration of gamma activity (40 Hz) were observed in mTBI subjects performing force production tasks 3 years post-injury [57]. More recently, we showed a significant reduction of EEG power within theta and delta frequency bands during standing postures in subjects with single and multiple concussions up to 3 years post-injury [58] and reduced amplitude of cortical potentials (MRCP) up to 30 days post-injury [60]. Unfortunately, there is no systematic EEG research available in the literature on subjects suffering and recovering from multiple concussions.
We applied advanced EEG–wavelet entropy measures to detect brain functional deficits in mTBI subjects. These EEG measures were significantly reduced after the first and more significantly after the second mTBI far beyond 7 days post-injury. Most importantly, the rate of recovery of EEG entropy measures was significantly slower after second mTBI compared to the first concussion [61]. Recently, we reported the alteration of EEG signals in mTBI subjects detected by a novel measure of nonstationarity, named Shannon entropy of the peak frequency shifting [62]. These findings are complementary to our previously published concussion report indicating the presence of residual deficits in mTBI subjects detected by multichannel EEG signals classifier using support vector machine [63]. We also conducted an EEG resting state study and reported the alteration of cortical functional connectivity in mTBI subjects revealed by graph theory, ICA, and LORETA analyses. Overall, a clear departure from small world-like network was observed in mTBI subjects [59].
The presence of a residual disturbance of the neuronal network is involved in execution of postural movement in mTBI subjects incorporating EEG- and VR-induced measures [64]. There was a significant increase of theta power during the progression of a balance task. Specifically, this theta increment was obvious initially at central areas with further diffusion to frontal electrode sites bilaterally. Interestingly, no significant theta power was present in concussed subjects at either phase of postural task progression. Most recently we reported that 85 % of mTBI subjects who showed significant alteration of alpha power in acute phase of injury did not return to pre-injury status up to 12 months [65].
Compensatory Approach During Concussion Assessment Batteries
Several studies have found electrophysiological deficits in asymptomatic concussed participants [66–68]. In these studies, concussed participants displayed normal levels of cognitive functioning, yet continue to show physiological dysfunction on EEG measures. The authors cite an unknown compensatory mechanism as an explanation for the findings. As part of our research, we sought to investigate this compensatory mechanism in more detail. In order to assess this, we chose to record EEG signals while participants were completing clinical concussion assessment measures, specifically the Immediate Post-Concussion Assessment and Cognitive Testing (ImPACT) neuropsychological and VR balance and spatial navigation modules, as well as EEG resting state evaluations in order to highlight the differences between clinical cognitive and balance performance and neuroelectric measures.
In a sample of 13 normal volunteers and seven concussed participants, no differences were found between groups on ImPACT and VR composite outcome scores. When looking at sub-scores, the only significant difference was poorer stationary balance in the concussed group. However, several significant group differences were found when looking at the EEG variables. For EEG resting state and ImPACT conditions, the concussed group had significantly lower power in the theta and beta bandwidths. Additionally, the concussed group had significantly lower alpha power during the ImPACT conditions and significantly lower delta power in the VR conditions. Conversely, the concussed group displayed significantly higher levels of coherence during EEG resting state and ImPACT evaluations, but lower levels of coherence during VR balance and spatial navigation testing.
Overall, for EEG resting state and ImPACT, these results indicate that concussed participants could not establish enough local effort (seen via lower power), so they recruited additional long-distance network connections (seen via the increased coherence). By recruiting additional networks, the concussed participants were able to successfully compensate for their neuroelectric deficits and produce normal clinical results. For the VR modules, concussed participants were not able to compensate as successfully. This was seen via the inability to recruit additional long-distance networks and clinically in the overall poorer balance in the concussed group, specifically the significantly worse static balance. This research indicates a disconnect between cognitive and neuroelectric resolution. Future research projects aim at determining whether cognitive functions resolve before physiological function or if current clinical concussion assessments are not sensitive enough to detect the residual affects of concussion.
Return to Play and EEG Concussion Research
One specific area that is still lacking in research and is noted in second international consensus statement [69] and in the statement from the World Health Organization’s (WHO) task force on mTBI [70] is the area of return to work or play. The WHO task force found no studies that demonstrate acceptable evidence to suggest when a person or athlete may safely return to work or the athletic field. As it has demonstrated its ability to identify physiological differences in the recovery from TBI, EEG should be considered as a feasible diagnostic tool for use within the “Return to Play” protocol and recommendations given to athletes returning to activity and sport.
As previously mentioned throughout this chapter, EEG has been used to study concussion or mTBI throughout all stages of recovery from acute, subacute to chronic or long term. One such clinical stage EEG has not been used is within the “Return to Play” stepwise progression back into athletic participation. The “Return to Play” protocol is the internationally accepted method for the safe return to activity of an athlete recovering from concussion [71]. This formalized “Return to Play” protocol has been in place since the original 2001 Concussion in Sport Group (CISG) Consensus Statement and remains unstudied and based on little to no scientific evidence, yet is still used as the “gold standard” for returning athletes to competition.
Under this procedure, “Return to Play” after a concussion follows a stepwise progression of increasing efforts and risk as outlined in Table 5.2. First the athlete must be completely asymptomatic at rest for a period of at least 24 h. Once asymptomatic and cleared by a supervising physician, the athlete may progress to a light aerobic exercise such as walking or stationary cycling. This light aerobic challenge is limited by restricting athletes to <70 % of their calculated maximum heart rate.
Table 5.2
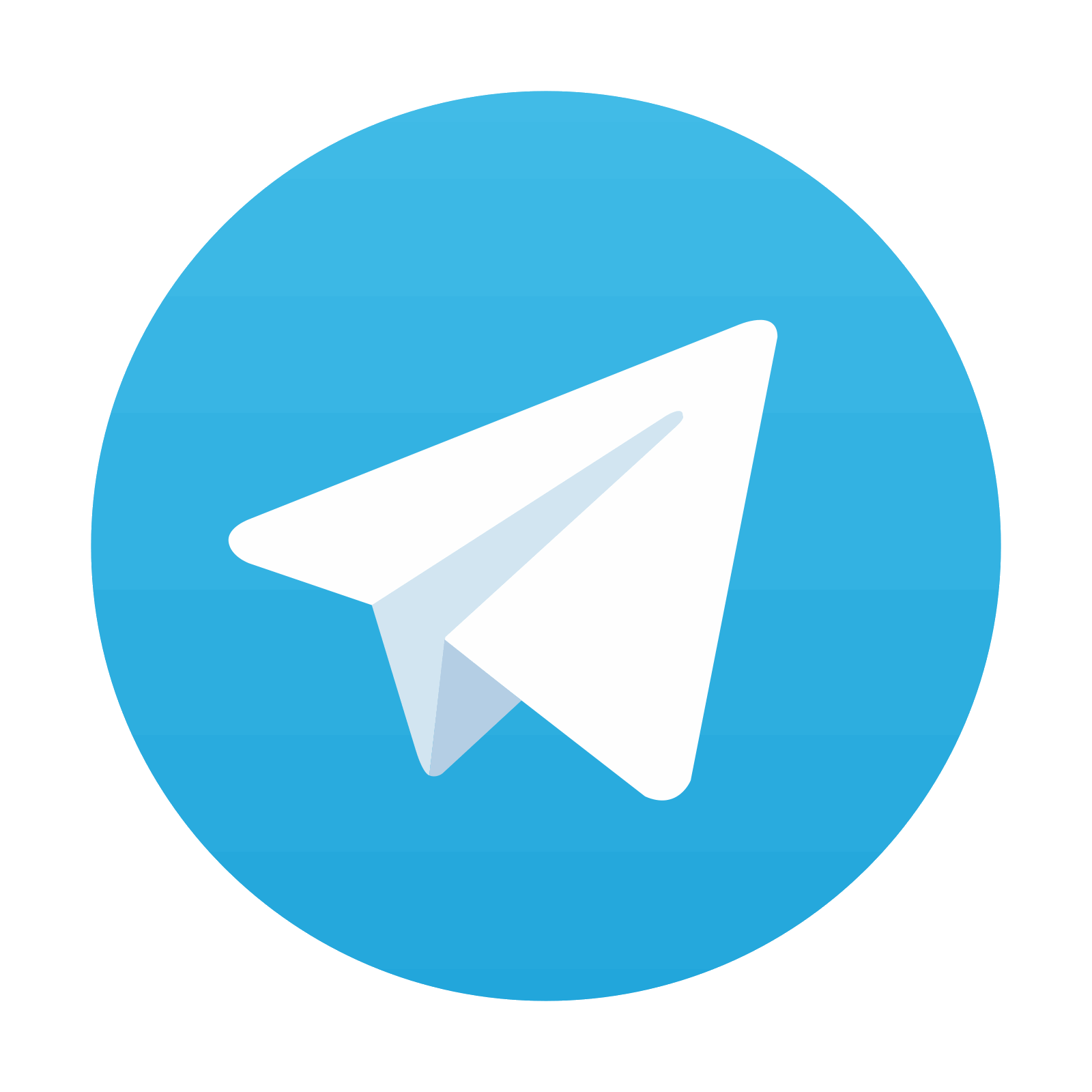
Graduated “Return to Play” protocol
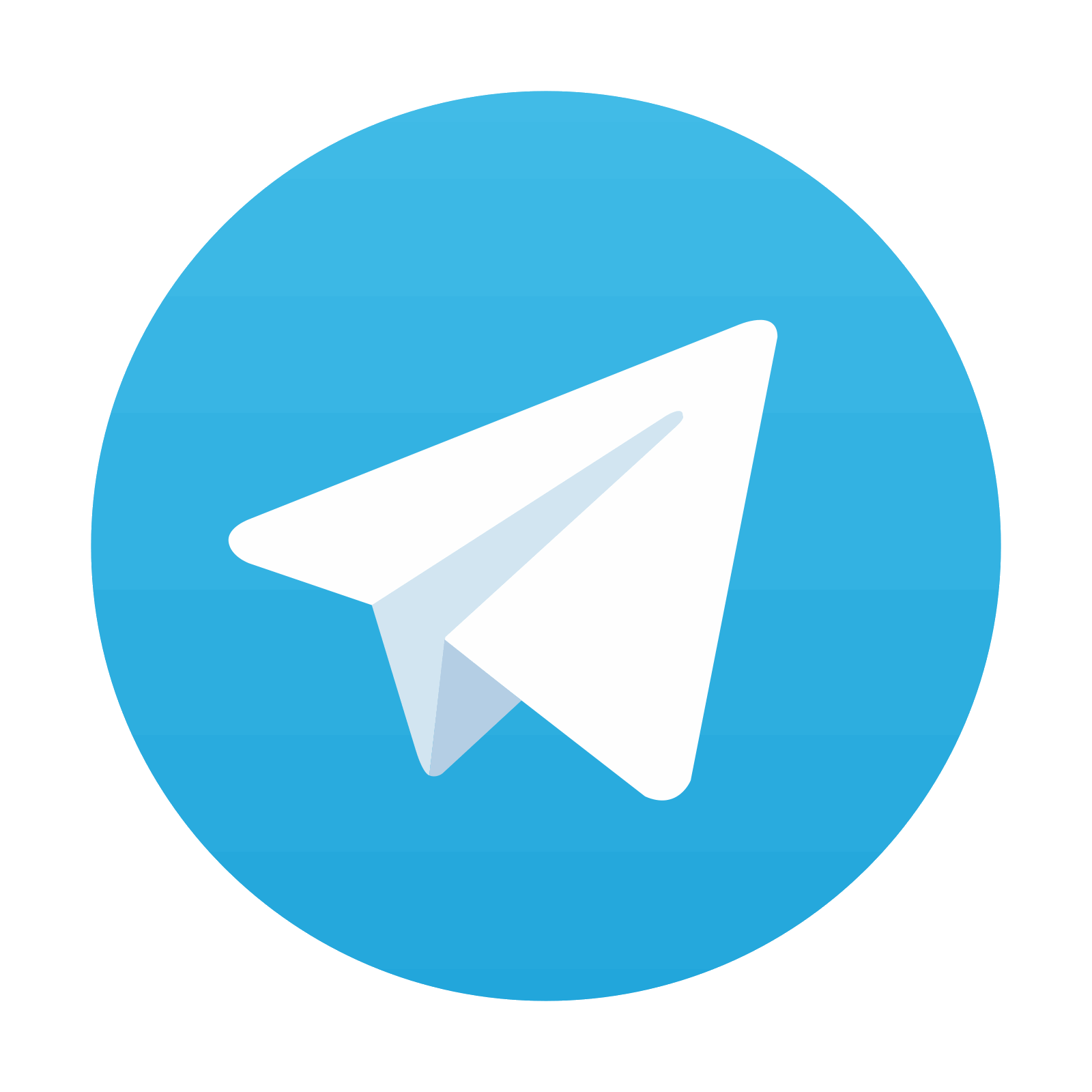
Stay updated, free articles. Join our Telegram channel
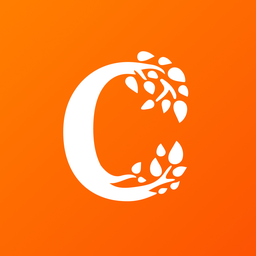
Full access? Get Clinical Tree
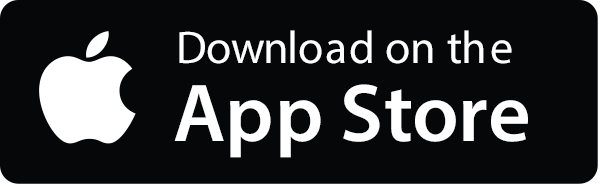
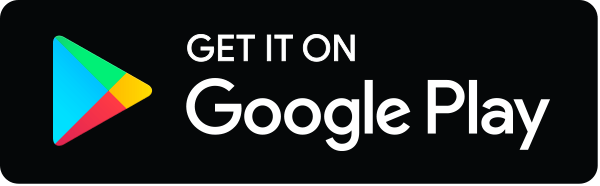
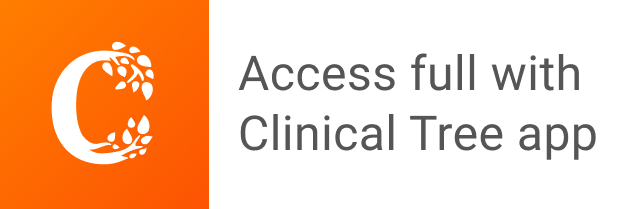