CHAPTER 16
Emerging Technologies in the Management of Upper Motor Neuron Syndromes
Ira G. Rashbaum and Steven R. Flanagan
Diseases and trauma of the central nervous system (CNS) afflict a staggering number of individuals of all ages and often result in lifelong disabilities. To comprehend the scope of the problem, one need only to examine the incidence of a single condition such as stroke, which afflicts 780,000 individuals each year in the United States and is the single most common cause of disability (1). Add to that the incidence of all other conditions affecting the CNS, including both traumatic brain and spinal cord injuries that occur at alarmingly high rates in younger individuals, it becomes clear that efforts to better delineate CNS pathology and reduce its morbidity must be a priority for researchers and clinicians.
In the past, CT and conventional MR technology permitted imaging of only macrostructural details of the living brain and spinal cord. Methods to examine their in vivo microscopic architecture and physiology were extremely limited, hindering researchers in their attempt to better delineate the complexity of both normal and pathological functioning. Consequently, the development of more effective treatment modalities was hampered. However, recent advances in imaging technologies have substantially increased our knowledge and understanding of both normal and pathological CNS functioning. This enhanced knowledge will be used to better delineate the pathology that results from injury as well as the recuperative responses that ensue, providing clinicians with the clues needed to develop more effective treatments. These advances also offer researchers the opportunity to further assess the changes in the maturing brain that account for developing skills occurring throughout early childhood, adolescence, and adulthood; the adaptive responses to disease and trauma; and the physical interactions among various cerebral regions that underlie normal human function.
Advanced treatment modalities, such as transcranial magnetic stimulation (TMS), virtual reality (VR), electrical stimulation (ES), robotic therapy, constraint-induced movement therapy (CIMT), and body weight–supported treadmill training (BWSTT), are currently being investigated as means to enhance recovery. They incorporate knowledge gained from research and advancements in neuroimaging that have enhanced our understating of neural plasticity and the role it plays in recovery. Advances in technology will demonstrate changes in the management of muscle overactivity in two separate yet complementary manners. The first is in the area of improved methods of diagnostic evaluations, whereas the second will address changes in the treatment modalities available for the clinicians in their clinical efforts. This chapter addresses advancements in both of these areas as advances in technologies that will likely lead to a more fundamental understanding of the CNS, and amelioration of morbidity caused by disease and trauma are mentioned in the discussion that follows.
Although many of the technologies discussed are currently used for research purposes, they hold the possibility of providing clinicians valuable information regarding disease processes and outcomes. First, they provide in vivo information pertaining to pathology and physiology that impacts function. Enhanced understanding will help researchers develop new means to treat impairments associated with upper motor neuron disorders. This new knowledge can also be used in conjunction with traditional therapies and to aid in developing new technologies to improve rehabilitation outcomes. Finally, they will help guide treatment and predict outcomes that will likely be useful to provide care that is both effective and efficient.
NEUROIMAGING TECHNOLOGIES
Standard CT and T1/T2-weighted MR images of the CNS provide valuable information regarding macrostructural abnormalities arising from trauma and disease. Unquestionably, these technologies have enhanced our ability to treat people in more efficient and definitive ways not feasible before their clinical implementation. However, in the adult human brain, white matter on both CT scans and T1/T2-weighted MR images appear homogeneous despite its inherent complexity. They also cannot adequately assess microscopic lesions such as those associated with diffuse axonal injury or changes in either metabolism or the concentration of biological molecules occurring in specific disease states. Recent advances in imaging technologies are enabling researchers and health care providers to noninvasively peer into the living brain, enhancing our knowledge of the structure and function of the CNS that will ultimately improve our ability to diagnose and treat people with neurologic disabilities.
Diffusion Imaging
Diffusion imaging uses MR technology to detect the movement of water molecules, providing a means to visualize cerebral tissue based on both the speed and direction of its movement. The manner in which water diffuses in biological tissue is impacted in specific ways, such as by the presence and chronicity of particular disease states. For example, restricted diffusion occurs in acutely infarcted cerebral tissue, but later increases as the stroke becomes chronic, offering a sensitive means to differentiate acute from chronic stroke. This can be used clinically when combined with findings from cerebral perfusion studies. After very acute stroke, diffusion images rapidly indicate the area of infarct and theoretically nonsalvageable tissue. Perfusion images identify regions of impaired blood flow, which after very acute stroke is often larger than the region of diffusion abnormality. This difference between diffusion and perfusion abnormalities, referred to as the diffusion–perfusion mismatch, is thought to represent the penumbra, the region of the brain susceptible to infarct if restricted blood flow is not restored. Results of several studies have indicated that thrombolytic therapy may be helpful in reducing stroke volume and improving clinical outcomes when given up to 6 hours after stroke onset, rather than just the currently approved 3-hour window when there is a significant diffusion–perfusion mismatch (2,3). Improved clinical outcome appears to be more likely in those with mismatch and isolated middle cerebral artery occlusions as opposed to those with concomitant middle cerebral and internal artery occlusions (4).
Diffusion tensor imaging (DTI) takes advantage of the fact that water diffuses faster along the parallel orientation of axons and fiber bundles as opposed to across them, properties referred to as anisotropy and isotropy, respectively. Water diffusion is inhibited perpendicular to fiber tracts because of restrictions imposed by the axolemma, myelin, and neurofilaments. However, it is relatively unrestricted along the path parallel to the orientation of the tract. Therefore, the degree of anisotropy, typically referred to as fractional anisotropy (FA), is high in well-organized white matter tracts where water moves rapidly parallel along the orientation of the bundle. Images are derived on the basis of the magnitude of FA as well as the orientation of the fibers within a tract, providing structural information pertaining to the integrity of white matter. For example, in cases of traumatic axonal injury where standard neuroimages are often unremarkable, DTI can image disruption of white matter tracts. DTI also provides a means to image the direction of fiber tracts by color coding them according to their orientation: typically red for left–right, green for anterior–posterior, and blue for cranial–caudal directions (5). Although color coding is useful, it is two dimensional, which limits its ability to assess integrity along an entire fiber tract. Three-dimensional tractography overcomes this by assessing a tract along its entire length. This offers a means to better assess both the function and integrity of individual fiber tracts that can be used to better understand the function and connectivity of cerebral structures, to examine the impact specific lesions have on motor function, and image damage to white matter not feasible with standard imaging.
Although DTI is becoming more widely accepted as a means to assess the degree of axonal injury after traumatic brain injury (TBI), it remains a research tool and is not used clinically at this time. It is effective in imaging axonal disruption in the presence of normal-appearing standard MR images (6), the extent and quantity of white matter injury (7), and the location of white matter tract injury associated with motor impairment (8). DTI data have also been correlated with both TBI severity and outcomes (9–11) and have been demonstrated as an effective tool to predict motor outcomes poststroke. After stroke, FA decreases as the time from the infarct increases. As Wallerian degeneration progresses after stroke in long white matter tracts, DTI detects decreased FA in sites distal but previously connected to the site of injury. The magnitude of FA reduction in the pyramidal tracts has also been correlated with motor outcomes poststroke, providing a potential means to predict outcomes (12).
Magnetic Resonance Spectroscopy
Magnetic resonance spectroscopy (MRS) determines the relative concentrations of various molecules in specific cerebral regions, which provides information regarding the chemical makeup of the brain in both healthy and disease states. Data are presented as a graph depicting the concentrations of various compounds, which are then typically presented as a ratio of one compound to another. Information can be obtained from either a single volume of brain, known as single voxel spectroscopy, or as a two-dimensional or three-dimensional analysis obtained simultaneously over wider regions, referred to as MRS imaging. Several compounds of interest measured by MRS include N-acetylaspartic acid (NAA), creatine, choline, myoinositol, glutamate, and lactate. NAA is located only in the neuronal tissue, with relative decreases in its concentration suggesting neuronal injury. MRS has detected reduced levels of NAA after TBI even in the presence of normal-appearing standard imaging (13), with evidence indicating it correlates with outcomes (14–16). Determining the relative concentrations of other compounds provides additional information, as detailed in Table 16.1, which can be used clinically in a number of ways. For example, MRS has been found to be a sensitive means of differentiating neoplastic from nonneoplastic lesions by determining the ratio of NAA to choline, which is typically reduced in neoplastic as compared to nonneoplastic lesions (17–22). MRS data have also been found to correlate with several poststroke outcomes, including motor recovery (23), apathy (24), and depression (25). For example, during the first several months after acute stroke, increasing NAA/Cr in the contralesional hemisphere has been found to be associated with improved neurologic status (23).
Functional MRI
Functional MRI (fMRI) is a technique that images cerebral regions that are activated during specific motor, sensory, or cognitive activities. It generates images by assessing the combination of physiological changes associated with metabolic activity, including localized alterations in blood flow and the levels of various forms of hemoglobin. fMRI detects the difference in magnetic properties of oxygenated and deoxygenated hemoglobin, which is altered by the combination of metabolic activity and changes in local blood flow that result from regional activation. The blood oxygen level difference (BOLD) response is represented as a bright signal on fMRI, indicating a region that is more metabolically active than surrounding tissue. Its temporal and spatial resolutions are good, both of which are better than those obtained by either single-photon emission CT or traditional positron emission tomography scans. An additional advantage over positron emission tomography and single-photon emission CT is the lack of radiation exposure associated with fMRI that permits for multiple imaging. Like all functional imaging studies, several factors must be considered when interpreting fMRI data. These include a subject’s ability to cooperate in a tightly confined MR scanner, medication effects on cerebral activation, adequacy of cerebral blood flow, and inadvertent subject movement during image acquisition. Data interpretation is complex in that it is important to consider the multitude of possible reasons accounting for specific activation patterns. For instance, does an activation pattern in a subject with brain injury differ from a normal control because of the establishment of alternative pathways, practice effects, or differences in performance difficulty that exist between injured subjects and normal controls? Other important issues to consider during interpretation include lesion location and size, time postinjury, subject age, the time postinjury the data are obtained, the subject’s cerebral dominance, previous injury, presence of cerebral vascular disease, and type of study design (eg, cross-sectional vs longitudinal). fMRI has provided valuable insights into both normal and pathological cerebral physiology, although there are limitations to its use. Although not extensively used to assess cortical changes associated with spasticity, limited fMRI evidence revealed cortical changes manifested as decreased activation in the hemisphere contralateral to the spastic limb injected with botulinum toxin, particularly in the posterior cingulate/precenous regions in four subjects with poststroke spasticity (26). The type of activity performed in MRI scanners is restricted, which prevents it from studying many complex real-world activities and limits its ecological validity. It is also subject to the same contraindications as standard MRI. At the present time, it is used as a research tool, although it has potential as a means to guide treatment and assess treatment effects.
TABLE 16.1
COMPOUNDS DETECTED BY MAGNETIC RESONANCE SPECTROSCOPY AND THEIR MARKERS | |
Metabolite | Marker |
NAA | Neuronal marker |
Creatine | Energy metabolism marker |
Choline | Cell membrane disruption, inflammation, and changes in myelination |
Myoinositol | Astrocyte marker |
Lactate | Metabolism |
NAA, N-acetylaspartic acid.
Functional Near-Infrared Spectroscopy
Functional near-infrared spectroscopy (fNIRS) is a technology that, like fMRI, determines the presence of cerebral activity by assessing the different concentrations of oxygenated and deoxygenated hemoglobin associated with metabolic activity. However, rather than assessing the magnetic differences of the two compounds, fNIRS assesses the differences in their optical properties. The system uses light sources placed on the scalp using a wearable device. The light passes relatively easily through the scalp and skull and into the top few millimeters of the brain. Light sensors on the scalp detect wavelengths of light that are reflected back to the surface. These sensors can detect the differences in near-infrared wavelength absorption of oxygenated and deoxygenated hemoglobin reflective of local metabolic activity. It has demonstrated ability to assess cerebral activity in the human cortex associated with both motor and cognitive tasks (27). It has been used to study various changes in metabolic patterns in subjects with dementia (28–30), schizophrenia (31), and stroke (32–36). It has several advantages over fMRI in that it is less susceptible to motion artifact as the sensors move with the subject, and it is considerably less expensive. It is also not limited by the same contraindications of fMRI, such as the presence of metal plates and subject claustrophobia. Perhaps most importantly, it is a portable system that can assess changes in cerebral activation during tasks performed in settings more ecologically valid for a particular function. This is not feasible in an MR scanner. For the same reasons, it can also assess activation patterns occurring during specific rehabilitation interventions. It therefore offers excellent temporal resolution that may provide opportunities to better develop and assess specific treatment interventions. It is becoming a more accepted technology now that it has been correlated with fMRI (33,37,38). Despite its utility, it is limited by its inability to image beyond the top 2 to 3 mm of cerebral cortex and 1 cm lateral to the topical sensors. As a result, it may be efficacious in evaluating the motor system of the upper extremity, but cannot be used to evaluate the lower extremity. In addition, unlike fMRI, cranial reference points are used to correlate activity with specific cerebral regions, decreasing its spatial specificity. Other problems include difficulties regarding the reduction of optical signals by the noncerebral tissue, the impact of pigmentation on signal detection, and the limited spatial resolution (39).
EMERGING TREATMENTS
Constraint-Induced Movement Therapy
CIMT has emerged as a therapeutic approach for selected stroke survivors with plegic upper limbs. It was described originally as an intensive therapeutic regimen provided over the course of 14 days that restricts the use of the uninvolved upper limb, forcing individuals with stroke to use their plegic limb to perform activities of daily living (ADL). It is based on the theory of learned nonuse, which states a limb weakened by stroke will not improve if it is not actively rehabilitated. For example, during the course of rehabilitation, individuals are taught to walk using their unimpaired and plegic legs, as ambulation requires the use of both lower limbs. However, because many ADL can be performed using only the uninvolved upper limb, there may be reduced rehabilitation efforts directed at improving the use of the plegic arm and hand because the primary focus is often to quickly return an individual to independent function. In this scenario, individuals with stroke are taught not to use their plegic upper limb, a process referred to as learned nonuse. Although compensatory one-handed strategies are useful to quickly improve the performance of specific activities, it does not result in cortical reorganization that leads to enhanced use of the plegic limb. However, CIMT has been shown to improve the use of stroke-weakened limbs in association with cortical changes (40).
There are several critical factors involved in CIMT, including intense, repetitive practice using the plegic limb for common functional tasks. This is accomplished in conjunction with physical restriction of the unimpaired arm and hand for up to 90% of waking hours through the use of a mitt, sling, and/or splint. A therapeutic technique, known as shaping, that trains the plegic limb to move through successive approximations of a desired task is performed. Traditional CIMT is extremely intensive and involves at least 6 hours of daily therapy for 2 weeks. The most comprehensive study examining its effectiveness compared to usual rehabilitation care is the Extremity Constraint Induced Therapy Evaluation (EXCITE) trial. It included 222 patients with a single stroke sustained 3 to 9 months before the time of enrollment. Compared to the usual treatment group, those receiving CIMT had less self-perceptions of hand function difficulty and performed better on several tests of upper limb function (41). An update from the EXCITE trial demonstrated the same level of significant arm motor functional improvement in patients 15 to 21 months after stroke (42).
An interpretation of the EXCITE trial by Dobkin (43) noted that a formalized care strategy for the usual treatment group would have helped clinicians critically interpret the value of CIMT, as the CIMT group may have been biased in its self-appraisal. In addition, a short phase-in of therapy for all subjects could have established whether the subjects were reasonably stable in their affected arm function before randomization, and an interim measure would have partially addressed whether less-intensive CIMT could work as well as traditional CIMT (43). Challenges imposed by the intensity and time commitments of CIMT have led the evaluation of several modifications in the way it is delivered. For example, modified constraint-induced therapy provides treatment 5 hours daily for 5 days followed by 3 hours a day, three times weekly over approximately 10 additional weeks. Results using this approach were comparable to traditional CIMT, indicating that alternative methods can be successfully used (44). Modified CIMT consisting of 6 hours of daily therapy for 2 weeks in subjects with chronic poststroke spastic hemiplegia resulted in reduced spasticity and improved upper limb function that persisted for at least 6 months (45). Additionally, it has been demonstrated that the extent of improvement from CIMT does not depend on the anatomic location of the stroke (46). A systematic review provided fairly strong evidence that modified CIMT, compared to traditional rehabilitation, could reduce the level of disability and improve the ability to use the paretic upper limb (47). Other modifications have used online computer sessions or combined CIMT with robotic therapy. In one pilot study, subjects with chronic stroke utilized online therapy sessions with computer-based cameras as they restrained their less-impaired upper limb resulting in functional improvement after 10 weeks of treatment (48).
There are other factors that limit the effectiveness and application of CIMT. It requires considerable effort and motivation on the part of those receiving it, with some evidence indicating that compliance with the mitt restriction to be as low as 32% (49). Ideally, subjects should not be at risk for falls because restraint of the more functional upper limb may prevent the effective use of walking aids, thus predisposing some individuals to injury. Another important factor restricting its use is the relatively small percentage of individuals with stroke for whom it has shown to benefit. Most trials have included only those individuals with active extension of at least 10° at the fingers and 20° at the wrist, markedly limiting the number of individuals who can benefit from its use.
Challenges ahead for CIMT include clarification of how much of the motor and functional gains are derived from the intervention versus the intensity of treatment and whether modifications in dose intensity impact outcomes. This is an important factor when considering if it is financially feasible in current and future economic environments.
Virtual Reality
Development of computer technology has led to the creation of enhanced VR programs that permit individuals to interact in computer-generated environments that simulate real-world settings (50,51) for both clinical and research purposes. It is a modality that uses technology to create virtual environments where feedback, intensity, and duration can be modified and used in settings that are more controllable than natural environments. In this scenario, specific therapies can be safely provided that may otherwise be too dangerous or complex in actual settings. VR environments can therefore reduce concerns over the consequences of allowing a person with physical or cognitive impairments to perform potentially dangerous activities on his or her own. The technology is extremely flexible, capable of remaining completely consistent over infinite repetitions or altering the type and pattern of sensory inputs and task complexity that can meet a multitude of clinical, research, and assessment needs. Not surprisingly, it has been gaining wider use in rehabilitation settings for both assessments and treatments.
VR has been shown to be a useful modality to improve functional skills after stroke. Jaffe et al (52) developed a head-mounted device worn like a hat displaying virtual objects. Patients wear it while walking on a treadmill, with a harness attached. Movement of lower extremities is promoted by negotiating virtual objects obstructing the path. When a virtual object is not successfully avoided, a virtual collision occurs that results in feedback to the patient. Performance has been shown to improve with additional VR training sessions, resulting in fewer “collisions.” This form of VR rehabilitation resulted in improved performance on an actual obstacle course compared to conventional ambulation training (52). Fung et al (53) applied a treadmill mounted on a platform that interfaced with a rear projector. The projector simulated a walking environment while the subject received auditory, sensory, and visual feedback. Subjects with stroke who were trained on this system were noted to have increased walking velocity (53). Improvement in ambulation has also been demonstrated by combining VR with robotic therapy. Deutsch et al (54) used a desktop computer combined with a seated system that allowed a subject to use his or her own ankle movements to operate a foot pedal. The subjects were instructed to use the foot pedal to “navigate” a virtual boat or plane while they received visual, auditory, and sensory feedback. Improvements were noted in gait velocity and endurance after 12 hours of training provided over more than 4 weeks (54).
Jang et al (55) investigated cortical reorganization and associated functional recovery using VR in patients with chronic stroke. VR was used to simulate tasks such as lifting crates and making saves as a soccer goalie. Cortical activation and associated motor recovery were measured before and after the intervention with fMRI and clinical tests assessing motor function. Before the intervention, bilateral primary sensorimotor cortices, contralesional premotor cortex, and ipsilesional supplementary motor areas were activated. After training, the activations in the contralesional hemisphere diminished while ipsilesional sensorimotor activation predominated in association with improved motor function (55). Similar findings of enhancing activation in the lesioned hemisphere after the use of various VR-related therapies suggest it may be a useful modality to induce cerebral plasticity and improve motor skills after stroke (56). Sin and Lee studied 40 hemiplegic stroke patients in a randomized controlled trial of VR using the Xbox Kinect with significant upper limb functional improvement that may have been a result of the greater total intervention time in the training group (57).
Limited studies have compared VR’s effectiveness to therapy provided in a real environment (58). In addition, the potentially synergistic effect of combining different technologies, such as with robotic therapy or cortical brain stimulation with VR, has received only limited attention (59). Lee and Chun piloted a randomized controlled trial of transcranial deep current stimulation with VR in patients with subacute stroke and found that the combination of these could facilitate a stronger beneficial effect on upper limb impairment than using either intervention alone (60).
VR-based rehabilitation for motor, ADL, and cognitive training shows promise, yet additional research is necessary to verify and refine preliminary study findings. For example, only limited studies have compared its effectiveness to therapy provided in a real environment (58). In addition, the potentially synergistic effect of combining different technologies, such as robotic therapy and VR, has received only limited attention (59).
Transcranial Magnetic Stimulation
TMS is a noninvasive technology that can both enhance and inhibit focal brain activity, potentially inducing cerebral plasticity and enhancing recovery after injury. TMS uses short magnetic pulses that are generated by passing a very brief electric current through a stimulating coil, typically made of copper encased in plastic that is held to the scalp’s surface. The magnetic pulse enters the brain after passing through the scalp and skull, where, depending on the pattern of pulse provided, it results in either an increase or decrease in cortical excitability. Therefore, unlike electric stimulation, which excites neurons directly, TMS stimulates neural tissue indirectly by inducing electrical activity via magnetism. The size and extent of the magnetic pulse depend on the spatial configuration of the stimulating coil and the position in which it is held. Current applications of TMS after brain injury include measuring the excitability of central motor pathways, mapping cortical representations, and predicting motor recovery (61–63), whereas other potential uses include enhancing cognitive and motor function resulting from brain injury.
TMS can be delivered via several patterns, including single-pulse, paired-pulse, and repetitive stimulation (rTMS). TMS delivered in the form of single-pulse stimulation over the motor cortex provides information pertaining to corticospinal tract excitability by measuring the motor response in the muscle corresponding to the area of the brain being activated. Paired-pulse stimulation can elicit measurable cortical inhibition or facilitation. These paired pulses are delivered through one or two magnetic coils at varying intervals to elicit either inhibition or facilitation. Repetitive TMS applies a repeated train of magnetic pulses at either low (1 Hz) or high frequencies (5–20 Hz) that cause suppression or enhancement of cortical excitability, respectively (64–66). Repetitive TMS can induce changes in excitability that last beyond the application of the magnetic pulses (67), suggesting that it has the capacity to induce long-term potentiation (68) that may be conducive to cerebral plasticity.
Repetitive TMS alters cortical excitability noninvasively and can be used in distinct ways to better understand cerebral physiology and function. For example, activation in a focal brain region can be inhibited, thereby inducing a temporary “virtual lesion” in an otherwise healthy tissue, resulting in the altered performance of a specific task. This provides information regarding the role of that particular cerebral region in the performance of that skill. Alternatively, activation can be enhanced, which again may impact the performance of a motor or cognitive task. This can potentially lead to a more thorough understanding of both cerebral physiology and the neuroanatomical correlates of impaired function resulting from disease or trauma (60–70).
Several studies have demonstrated the potential use of TMS as a means to enhance motor recovery after a stroke. For example, high-frequency repetitive TMS applied over the lesioned hemisphere, which enhances excitability, resulted in improved hand function of the impaired limb in subjects with acute stroke (71). Interestingly, many studies have examined the functional impact of inhibiting the uninjured hemisphere by applying low-frequency rTMS, which is typically overactive after stroke (72,73). The overactivity of the contralesional hemisphere that is observed during movement of the paretic limb (74,75) is felt to inhibit activation of the lesioned hemisphere, a process referred to as interhemispheric inhibition. Low-frequency rTMS applied over the primary motor cortex of the contralesional hemisphere, resulting in its inhibition, has been shown to improve the function of the affected hand after acute and chronic stroke in subjects of various ages through the process of transcallosal inhibition (76–80), that is, decreasing the activation of the contralesional motor cortex impairs its ability to inhibit the lesioned cortex, resulting in improved motor skills of the hemiparetic limb. fMRI data revealed that overall changes in cerebral activation patterns associated with rTMS-induced inhibition of the contralesional motor cortex appeared similar to normal patterns (81).
TMS may become a practical means to enhance recovery after acquired brain injury. As additional meta-analyses and summaries of trials have provided further evidence of its effectiveness (82–84). However, it should be noted that the extent of motor impairment at the time of treatment may influence the degree of motor recovery achieved using this technology (85).
The changes in cortical excitability induced by TMS have also been studied as a means to manage spasticity in several upper motor neuron conditions, including stroke (86–88), multiple sclerosis (89,90), cerebral palsy (91), and spinal cord injury (92–95). Corticospinal neurons are known to modulate a and g motoneurons, Ia afferent sensory fibers, and spinal interneurons, all of which are involved in the generation of spasticity. In general, increasing corticospinal tract excitability by using rTMS is theorized to inhibit overexcitability of a and g motoneurons, thereby reducing spasticity. Various rTMS paradigms have been tested with results indicating that it has considerable potential to either reduce spasticity (87,89,90) and/or increase passive range of motion (86,91), thereby an improve functional use of affected limbs caused by CNS lesions.
Another potential benefit of rTMS includes decreasing visuospatial neglect in acute stroke (96). The primary risk of rTMS has been thought to be its potential to induce seizures, which can be minimized by adhering to recommendations that limit stimulation parameters, advise monitoring guidelines, and detail contraindications (97). A meta-analysis of rTMS after stroke reported no rTMS-associated seizures (82). An additional risk may be hearing loss, particularly when sessions are provided regularly over several weeks. Potential uses of TMS after neurologic injury in the future will include enhancing our understanding of the physiology underlying the behavior by better delineating the mechanisms, locations, interactions, and adaptability of neuronal networks (98) and exploiting the effects of stimulation on cerebral plasticity to enhance functional recovery. Limitations of TMS include its inability to stimulate subcortical structures that restrict its impact to only those brain regions close to the scalp. The precise timing of when brain plasticity might best occur with TMS has not yet been determined, although as previously mentioned, it has shown promise at various periods poststroke. As opposed to TMS, repetitive peripheral magnetic stimulation appears not to impact motor function in subjects with severe hemiparesis, but may have a slightly favorable impact on spasticity (99).
Electric Stimulation
ES can be provided in several ways to improve both motor and functional skills after injury to the CNS. It can be applied to muscles or nerves in the form of neuromuscular electric stimulation (NMES) or to the brain via transcranial direct stimulation or epidurally placed electrodes. Neural plasticity occurs when motor learning is achieved by the performance of goal-oriented, highly repetitive active movement training, aimed at the improved use of a paretic limb. ES, either applied to muscles involved in a specific goal-oriented activity or to the motor cortex associated with the movement of paretic limbs being trained, provides a means to enhance motor relearning and theoretically improve the functional use of the paretic limb. NMES has also been studied as a means to reduce shoulder subluxation and its associated pain after stroke, as well as to reduce spasticity. ES enhances movement of paretic muscles incapable of sufficiently contracting on their own power, which when applied during highly repetitive training activities theoretically enhances motor relearning.
Clinical use of NMES can be provided in several ways. Cyclic NMES provides simulation at a set rate for a predetermined period of time. Electromyography (EMG)-mediated NMES detects electrical activity in a muscle that is generated by the intent to move it but is insufficient to contract it for functional activities. Once the device detects inherent muscle activity above a set threshold, it provides an externally generated electrical stimulus that causes the muscle to contract. It has a theoretical advantage over cyclic NMES in that it requires cognitive effort to move a limb, providing a biofeedback component that enhances its impact on neural plasticity. However, it is useful only in those muscles that an individual can at least partially activate on his or her own. NMES can also be provided to specific muscles in an appropriate time sequence to complete mobility or ADL tasks, such as to the ankle dorsiflexors during the swing phase of gait. This can be achieved by delivering stimulation by using either single-channel or multichannel units. However, as more muscles are stimulated for complex tasks, such as walking, which require the contraction of multiple muscle groups in a very specific pattern using multiple channels, the system becomes cumbersome and impractical. This is partially being addressed by developing percutaneous systems that will be detailed later.
NMES has been shown to fairly consistently improve motor function (100–109), strength (103,106,108–110), and use of paretic limbs for specific tasks (eg, lifting and moving objects) (106,108,109,111–113) for individuals with stroke at various times postinjury. Although its specific effect on motor relearning in the lower limb has been called into question (114), it has been found to be an effective means to improve gait speed (113,115,116,117), an important predictor of successful community ambulation. It has also been shown to be a more effective means to promote motor recovery than conventional rehabilitation techniques alone (100,103,104,106–109,111–113). However, the stability of results over time remains uncertain, as some studies documented sustained improvements for up to 9 months posttreatment (102,105), whereas others demonstrated that the benefits diminished as the time post-interventions passed (101,103). It also remains unclear which forms of ES are most useful, as different applications of NMES have not been directly compared to each other. It has been suggested that EMG-mediated devices have a theoretical advantage over cyclic systems (118), particularly when they are used to assist in completing functional tasks (97), which was supported by the results of a metanalysis (98). Despite improvements in motor skill and strength, additional studies will help to determine whether EMG-mediated devices are capable of improving quality of life or enhancing independent function after neurologic injury.
Transcranial direct current stimulation (TDCS) is a noninvasive brain stimulation technique that can be coupled with other therapies to potentially alter the stroke recovery trajectory by modulating brain activity noninvasively to induce plasticity. It is portable, which allows for simultaneous training with other therapies.
A weak direct current is provided by alkaline batteries attached to electrode pads applied to the scalp associated with a targeted cortical region. The reference electrode is placed on the contralateral supraorbital area or on the cheek or shoulder. Depending on the electrode size, the current density is approximately 0.04 to 0.07 mA/cm2 and is considerably below the safety threshold for neural injury. Commonly reported side effects include skin irritation with local scalp itching, tingling, or burning; transient headache; insomnia; skin lesions; and contact dermatitis.
TDCS modulates the excitability of targeted brain regions by altering neuronal membrane potential based on the polarity of the low-voltage direct current that is transmitted through the skull via the electrodes. The exact mechanism by which it modulates brain excitability to induce brain plasticity is not presently clear.
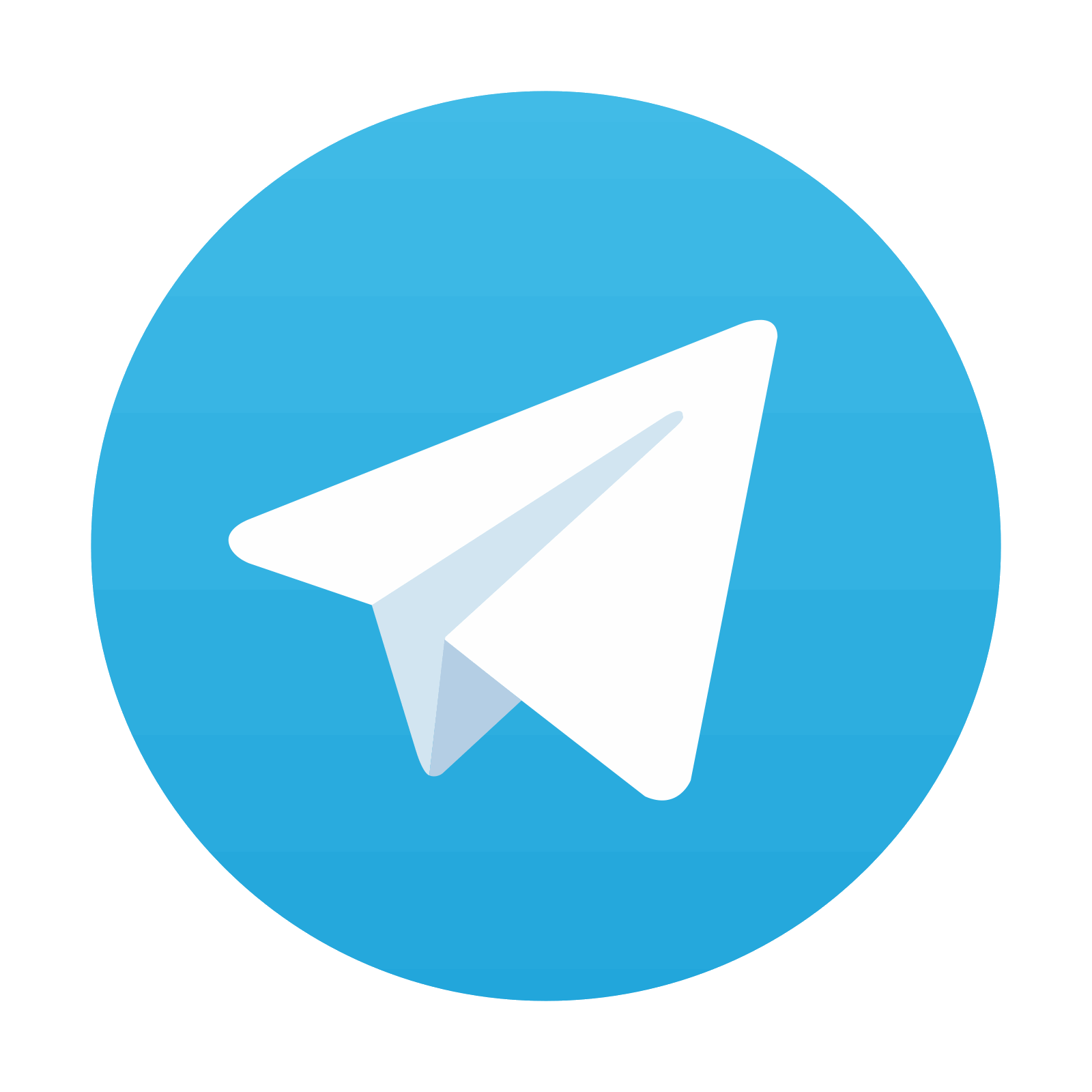
Stay updated, free articles. Join our Telegram channel
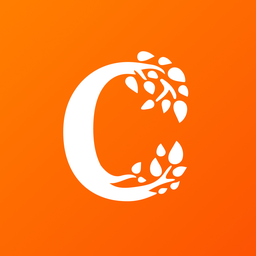
Full access? Get Clinical Tree
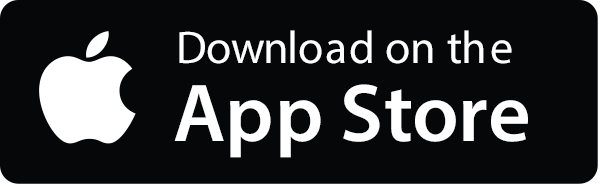
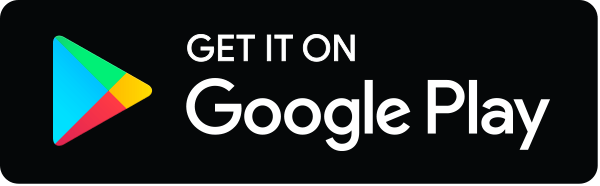