Electrophysiology
Jun Kimura
Electrical Properties of Nerve and Muscle
The same basic membrane physiology applies to both nerve and muscle, although different anatomic substrates subserve propagation of electrical impulses. The magnitude of the transmembrane potential in a steady state dictates the excitability of the tissues. Thus, understanding membrane physiology at the cellular level requires the knowledge of the ionic concentration of cell plasma and its role in maintaining transmembrane potentials. If an external stimulation depolarizes the cell to a critical level, called threshold, an action potential is initiated at the stimulus site, which then propagates across the membrane. The interstitial tissues act as a volume conductor when analyzing extracellular potentials by surface or needle electrodes in clinical electrodiagnosis. This chapter summarizes the basic physiology of the propagating action potential through volume conductors, which dictates the waveform of the recorded potentials.
Transmembrane Potential
The muscle membrane forms the boundary between intracellular fluid in cell cytoplasm and extracellular interstitial fluids. Approximately equal numbers of ions are dissolved in both compartments, but the cell is negative inside as compared to outside with a steady transmembrane potential (Em) of some -90 mV in the human skeletal muscle at rest (1). To maintain the steady-state equilibrium, the concentration gradient of potassium (K+), sodium (Na+), and chloride (Cl+) ions (Table 4-1) must counter this electrical force (Fig. 4-1). For example, the ionic concentration difference pushes K+ from inside to outside the cell, reflecting the higher concentration inside, whereas the negative equilibrium potential pulls the positively charged K+ from outside to inside the cell.
Table 4-1 shows the transmembrane potential of EK+ (-97 mV), ENa+ (+66 mV), and ECl+ (-90 mV) theoretically required to establish such equilibrium based solely on their ionic concentrations. These compare with the actual transmembrane potential (-90 mV) in the example under consideration. Thus, ionic concentration and transmembrane potential alone cannot maintain these ions in perfect balance. The other factors of import include selective permeability of the cell membrane to certain ions and the energy-dependent sodium-potassium (Na-K) pump.
In the case of K+, its inward transport by the energy-dependent Na-K pump makes up for the small discrepancy between EK (-97 mV) and Em (-90 mV). Here, electrical force (-90 mV) plus the pump transport (approximately equivalent to -7 mV) from outside to inside the cell counteracts almost exactly the concentration gradient (equivalent to -97 mV) from inside to outside the cell. Both the concentration gradient and potential difference (-90 mV) pull the Na+ from outside to
inside the cell, but only a small amount of the ion moves because of the impermeability of the cell membrane. Active transport of Na+ from inside to outside by the Na-K pump counters the small inward Na+ leak to maintain the equilibrium.
inside the cell, but only a small amount of the ion moves because of the impermeability of the cell membrane. Active transport of Na+ from inside to outside by the Na-K pump counters the small inward Na+ leak to maintain the equilibrium.
Table 4-1 Compositions of Extracellular and Intracellular Fluids of Mammalian Muscle (19) | ||||||||||||||||||||||||||||||||||||||||
---|---|---|---|---|---|---|---|---|---|---|---|---|---|---|---|---|---|---|---|---|---|---|---|---|---|---|---|---|---|---|---|---|---|---|---|---|---|---|---|---|
|
Generation and Propagation of Action Potential
As mentioned above, a voltage-sensitive channel regulates the conductance of Na+ and K+ ions across the membrane depending on the transmembrane potential. In the resting stage, K+ channels are open and Na+ channels are closed. An externally applied current induces negativity under the cathode, or negative pole, thus making inside the axon relatively more positive, or cathodal depolarization. Subthreshold stimulation produces a self-limited local change in the transmembrane potential that diminishes with distance. Threshold or suprathreshold stimulation depolarizes the membrane to a critical level by 15 to 25 mV from -90 mV to -65 to -75 mV in the human muscle cell (1). This degree of depolarization opens the voltage-dependent Na+ channels, causing a 500-fold increase in Na+ permeability, which initiates the sequence of events leading to nerve excitation.
The increased conductance or permeability allows Na+ to enter the cell, further depolarizing the cell, which in turn accelerates inward movement of this ion. This sequence results in dramatic change of Na+ permeability, with an explosive reversal of membrane potential from -90 mV to +20 mV. An action potential thus develops in an all-or-none fashion; that is, the same maximal response occurs regardless of the kind or magnitude of the stimulus (Fig. 4-2). In other words, a switch from the K+ to Na+ equilibrium constitutes the generation of an action potential. This negative-to-positive shift of intracellularly recorded membrane potential can be recorded as negative spike extracellularly, or upward deflection according to the convention of clinical electrophysiology.
With depolarization of membrane potential, permeability to K+ also increases, but only after a delay of about 1 ms. At about the same time, the increased Na+ permeability falls again to near the resting value with closure or inactivation of Na+ channels. Inactivated Na+ channels cannot be open for a few milliseconds even if the membrane is depolarized again above the critical level. This inactivation forms the basis of the refractory period. Inactivation of Na+ conductance, together with increased K+ permeability, results in a rapid recovery of the cell membrane from depolarization. After the potential falls precipitously towards the resting level, a transient increase in K+ conductance hyperpolarizes the membrane, which
then returns slowly to the resting state, completing the cycle of repolarization. The total amount of Na+ influx and K+ efflux during the course of an action potential is too small to alter the concentrations of these two ions in the intracellular and extracellular fluids.
then returns slowly to the resting state, completing the cycle of repolarization. The total amount of Na+ influx and K+ efflux during the course of an action potential is too small to alter the concentrations of these two ions in the intracellular and extracellular fluids.
An action potential initiated at one point on the cell membrane sets up an intracellular current that flows from the positively charged active area to the adjacent, negatively charged inactive regions. A return current flows from the inactive to active region through the extracellular fluid, completing the circuit (2). Thus, the local current enters the cell at the site of depolarization (i.e., sink) and passes out from the adjacent polarized regions on both sides of the active area (i.e., sources). When depolarization at the sources reaches the threshold, an action potential is generated, forming a new sink, which in turn gives rise to a new
local current in both distal and proximal directions. This results in orthodromic as well as antidromic volleys of the action potential from the original site of depolarization.
local current in both distal and proximal directions. This results in orthodromic as well as antidromic volleys of the action potential from the original site of depolarization.
![]() Figure 4-2 • Schematic diagram of graded responses after subthreshold stimuli and generation of action potentials after suprathreshold stimuli. Experimental arrangement shows intracellular stimulation (I) and recording electrodes (E) on top (A) and polarity, strength, and duration of a constant current on bottom (B). Hyperpolarizing (1) and subthreshold depolarizing current (2) induce a nonpropagating local response. Current of just threshold strength will produce either local change (3a) or an action potential (3b). Suprathreshold stimulation (4) also generates an action potential but with a more rapid time course of depolarization. (Reprinted from Woodbury JW. Action potential: properties of excitable membranes. In: Ruch TC, ed. Neurophysiology, 2nd ed. Philadelphia: WB Saunders, 1965:26–57 , with permission.) |
In an extracellular recording, an action potential consists of an initial negative spike of about 1 ms in duration, representing the intracellular positive spike of depolarization, and two subsequent after-potentials, depolarizing and hyperpolarizing (Fig. 4-3). The first externally negative deflection grafted onto the declining phase of the negative spike, a supernormal period of excitability, presumably represents sustained internodal positivity and the extracellular accumulation of K+ associated with the generation of an action potential. The subsequent externally positive after-potential, a prolonged subnormal period of excitability, reflects the elevated K+ conductance at the end of the action potential combined with an increased rate of the Na-K pump to counter the internal sodium concentration.
Anatomy and Physiology of the Neuromuscular Junction
The neuromuscular junction consists of the motor nerve terminal, junctional cleft, and muscle endplate. The release of acetylcholine (ACh) ensures unidirectional conduction from the axon terminal to the muscle endplate, similar to synaptic transmission in a sequence of neurons. Other characteristics of the chemical mode of transmission include a synaptic delay of a fraction of a millisecond and nonpropagating nature of postsynaptic potentials. Such a local potential causes no refractoriness, unlike the all-or-none response of the nerve or muscle action potential. Temporal as well as
spatial summation after subliminal stimuli provides greater flexibility and adaptability for graded responses. As in a synapse, the mobilization store must continuously replenish the liberated transmitter molecules, so that the neuromuscular junction would not fail due to depletion of immediately available molecules.
spatial summation after subliminal stimuli provides greater flexibility and adaptability for graded responses. As in a synapse, the mobilization store must continuously replenish the liberated transmitter molecules, so that the neuromuscular junction would not fail due to depletion of immediately available molecules.
Motor Endplate
The term “motor endplate” describes the postsynaptic membrane of the striated muscle, where the specialized motor nerve efferent endings terminate. Each muscle fiber usually has only one endplate, innervated by a branch of the motor axon. At the nerve terminals, Schwann cells without myelin (teloglial cells) separate the axon from the surrounding tissue. Thus, the neuromuscular junction consists of the motor nerve ending, Schwann cells, and muscle endplate (Fig. 4-4). After the nerve ending loses the Schwann cells at the junctional region, the axon terminal forms a flattened plate within a surface depression of the sarcolemma. This indentation of the muscle fiber, measuring about 200 to 500 Å deep, is called a synaptic gutter or primary synaptic cleft. The
thickened postsynaptic membrane in this region has a large number of mitochondria, nuclei, and small granules close to the narrow infoldings called the junctional folds or secondary clefts. Diseases of neuromuscular transmission alter the motor endplate profile (Fig. 4-5).
thickened postsynaptic membrane in this region has a large number of mitochondria, nuclei, and small granules close to the narrow infoldings called the junctional folds or secondary clefts. Diseases of neuromuscular transmission alter the motor endplate profile (Fig. 4-5).
Electron-microscopic studies of the human external intercostal muscles (3) show clear, round synaptic vesicles in the axon terminals, mostly clustered in the regions called active zones. The vesicles, containing ACh, are released into the synaptic cleft from this site. Approximately 50 synaptic vesicles are counted per square micrometer in a nerve terminal that occupies an area close to 4 μm+. The synaptic basal lamina, interposed between the nerve terminal and muscle cell, stores acetylcholinesterase (4). The postsynaptic membrane area is 10 times larger than the presynaptic membrane, forming elaborate junctional folds (5). The postsynaptic junctional folds containing a high concentration of ACh receptors cover an area about 2.5 times that of the terminal itself. The nicotinic ACh receptor, an ionotropic glycoprotein, comprises five subunits, α (alpha) ×2, β (beta), γ (gamma), and δ (delta) in the fetus and α × 2, β, ε (epsilon), and δ in the adult. Binding of two ACh molecules to the two α subunits initiates the opening of the ACh channel, allowing cations (predominantly Na+) to move through the postsynaptic membrane, with the net result of depolarization (6).
The presynaptic axoplasm stores the synaptic vesicles, which are intracellular structures 300 to 500 Å in diameter that encapsulate ACh molecules. The nerve endings also contain high concentrations of choline acetyltransferase, which synthesizes ACh, and acetylcholinesterase, which hydrolyzes ACh. The presence of the neurotransmitter and the two enzymes in the proximal portions of neurons suggests that enzymatic synthesis takes place in the cell body before they are transported to the nerve terminals (7). Each vesicle contains
a quantum (5,000 to 10,000 molecules) of ACh (8). A small portion of quanta (about 1,000) is located adjacent to the cell membrane for immediate release; many more (10,000) are contained in the mobilization store, which moves continuously toward the membrane to replace liberated ACh. The remaining and largest portion of quanta (300,000) forms the main store as a reserve supply for the mobilization store.
a quantum (5,000 to 10,000 molecules) of ACh (8). A small portion of quanta (about 1,000) is located adjacent to the cell membrane for immediate release; many more (10,000) are contained in the mobilization store, which moves continuously toward the membrane to replace liberated ACh. The remaining and largest portion of quanta (300,000) forms the main store as a reserve supply for the mobilization store.
Electrical Activity at the Endplate
Random release of a single quantum of ACh from the nerve terminal (9) induces a small depolarization of the postsynaptic membrane known as the miniature endplate potential (MEPP). The MEPP averages 1 mV in amplitude if recorded with a microelectrode inserted directly into the endplate region, but only 50 to 100 μV when registered with an ordinary needle electrode placed near the endplate of the muscle fibers. Each ACh vesicle contains a quantum, or a nearly equal number, of ACh molecules irrespective of external factors, thus maintaining the MEPP amplitude (a measure of quantum size) relatively constant. In contrast, the release of vesicles, and the occurrence of MEPPs, varies over a wide range in frequency, such as increasing with elevated temperatures.
Depolarization of the motor nerve terminal leads to the influx of calcium (Ca2+), enhancing quantal release by increasing fusion of the ACh vesicles with the nerve membrane. The resulting synchronized release of many ACh vesicles results in summation of MEPPs, giving rise to a localized endplate potential (EPP). The number of immediately available ACh quanta and the voltage-dependent concentration of Ca2+ within the axon terminal together determine the size of the EPP. The number of quanta emitted per nerve impulse, or quantum content, averages 25 to 50, based on the amplitude ratio of EPP/MEPP.
Like MEPPs, EPPs result from depolarization of the motor endplate by ACh. The opening of ACh receptors by the synaptic transmitter increases the conductance of positively charged ions, including Na+ and K+. The diffusion of these ions down their electrochemical gradients results in depolarization of the motor endplate. This nonpropagated local response begins about 0.5 ms after the release of ACh, peaks in about 0.8 ms, and decreases exponentially, with a half-decay time of about 3.0 ms. The EPP, a graded rather than all-or-none response, increases in proportion to the number of ACh quanta liberated from the nerve terminal and the sensitivity of the endplate to ACh molecules. It declines rapidly with distance from the endplate. Like the excitatory postsynaptic potential, two or more subthreshold EPPs, if generated in near synchrony, can summate to cause a depolarization exceeding the critical level for generation of an action potential.
When the EPP exceeds the threshold or the critical level of depolarization, a molecular change of the depolarized membrane results in a selective increase of Na+ conductance, followed by an increase in K+ conductance. As mentioned earlier, this phenomenon, inherent in the muscle membrane, occurs irrespective of the nature of the stimulus as long as depolarization reaches the critical value. The all-or-none characteristic of the amplitude is dictated by Na+ channel kinetics. In contrast, the speed of initial depolarization alters the latency of the action potential, which forms the source of jitter in single-fiber studies. A neuromuscular block results when the EPP fails to reach the critical level, either because of insufficient liberation of ACh vesicles from the axon terminal or reduced sensitivity of the muscle endplate. The generation of a muscle action potential is all-or-none for each muscle fiber, but the compound muscle action potential shows a graded response in proportion to the number of activated muscle fibers.
The muscle action potential, once generated at the endplate, propagates bidirectionally to the remaining parts of the fiber at a relatively slow rate of 3 to 5 m/s. The spread of action potential from the motor endplate to the transverse tubules initiates the excitation–contraction coupling, linking the electrical process to muscle contraction (10,11).
Potentials Recorded Through a Volume Conductor
During the clinical study, connective tissue and interstitial fluid act as a volume conductor surrounding the generator sources (2,12,13). Here, an electrical field spreads instantaneously from a
source represented as a dipole (that is, a pair of positive and negative charges). In a volume conductor, currents move into an infinite number of pathways between the positive and negative ends of the dipole, with the greatest current densities (the number of charges passing through a unit area per unit time) along the straight path. Analysis of electrical potential distribution in a volume conductor requires knowledge on pathways of current that generates potential gradients. Current path is described as current lines connecting both the current source and sink of the dipole. The more current lines penetrating a unit area (or the higher the current density), the steeper the potential gradient. In other words, isoelectric lines (points of identical unit potentials), which transect the current lines, become more crowded. Current pathways are predicted by a simple law that current tends to flow along the path of least resistance.
source represented as a dipole (that is, a pair of positive and negative charges). In a volume conductor, currents move into an infinite number of pathways between the positive and negative ends of the dipole, with the greatest current densities (the number of charges passing through a unit area per unit time) along the straight path. Analysis of electrical potential distribution in a volume conductor requires knowledge on pathways of current that generates potential gradients. Current path is described as current lines connecting both the current source and sink of the dipole. The more current lines penetrating a unit area (or the higher the current density), the steeper the potential gradient. In other words, isoelectric lines (points of identical unit potentials), which transect the current lines, become more crowded. Current pathways are predicted by a simple law that current tends to flow along the path of least resistance.
Characteristics of Current Flow
If the current flows in an infinite homogeneous volume conductor, a potential at a given point can be calculated mathematically; the potential is inversely related to the square of the distance from the dipole, and proportional to the cosine of the angle subtended by the point and the orientation of the dipole. By contrast, the human body provides a notoriously inhomogeneous and finite-shaped conductor. As a consequence, the analysis of current lines becomes extremely complicated and hardly predictable. Various tissues in the body have their own resistivity (Table 4-2) (14).
Table 4-2 Volume Conduction in Inhomogeneous Media (14) | ||||||||||||||||||
---|---|---|---|---|---|---|---|---|---|---|---|---|---|---|---|---|---|---|
|
The volume conductor has another characteristic of importance for determining the electrical potential. Capacitance is the ability of the conductor to store charge. If current flows in a volume, a portion of the current is used to store positive and negative charges of equal quantity. If capacitance is high, it takes longer to generate potential difference at a distance. If low, the same current produces a steeply rising potential. In the presence of a fixed capacitance, a quick build-up of current gives rise to a volume-conducted potential at more distant locations than a slow development of the same current (i.e., source currents with highly synchronized onset tend to generate greater volume-conducted potentials than those with gradual onset). Thus, capacitance serves much like a resistance in the situation with changing current. The term impedance is used to denote the total effective resistance acting against the changing current and includes the combined impact of resistance and capacitance.
Physiologic sources of current comprise those generated by both synaptic potentials and action potentials. Action potentials are a propagating source of current with front positivity. They are always drawn as current lines starting from this front current source and ending at the sink just behind, thus producing currents that are distributed widely. Potentials associated with these currents can be recorded from a distance in a volume conductor. Such potential fields are called open fields.
Some synaptic potentials in central nervous system nuclei make a spherical dipolar sink source. The central current sink is juxtaposed with the source surrounding it. In this case, current lines have a short path confined within the nucleus. As a result, no volume-conducted potentials are recorded at a distance. Such a configuration is termed a closed field distribution.
The current flow decreases in proportion to the square of the distance from the generator source. Thus, the effect of the dipole gives rise to voltage difference between an active recording electrode in the area of high current density and a reference electrode at a distance. Whether the
electrode records positive or negative potentials depends on its spatial orientation to the opposing charges of the dipole. For example, in an idealized homogenous volume, the active electrode located at a point equidistant from the positive and negative charge registers no potential, provided the reference electrode is indifferent. The factors that determine the amplitude of the recorded potential include charge density (the net charge per unit area), surface areas of the dipole, and its proximity to the recording electrode.
electrode records positive or negative potentials depends on its spatial orientation to the opposing charges of the dipole. For example, in an idealized homogenous volume, the active electrode located at a point equidistant from the positive and negative charge registers no potential, provided the reference electrode is indifferent. The factors that determine the amplitude of the recorded potential include charge density (the net charge per unit area), surface areas of the dipole, and its proximity to the recording electrode.
Diphasic and Triphasic Waveforms
With a pair of electrodes directly placed on the surface over a nerve or muscle, the nearest electrode (E1) becomes negative relative to the distant electrode (E2) as a propagating action potential reaches the recording site. This results in an upward deflection of the tracing according to the convention of clinical electrophysiology. The trace returns to the baseline at the point where the depolarized zone affects E1 and E2 equally. With further passage of the action potential, E2 becomes negative relative to E1, or E1 becomes positive relative to E2. Thus, the trace now shows a downward deflection until it returns to the baseline as the nerve activity becomes too distant to affect the electrical field near the recording electrodes. This produces a diphasic action potential, as shown in Figure 4-6 (15).
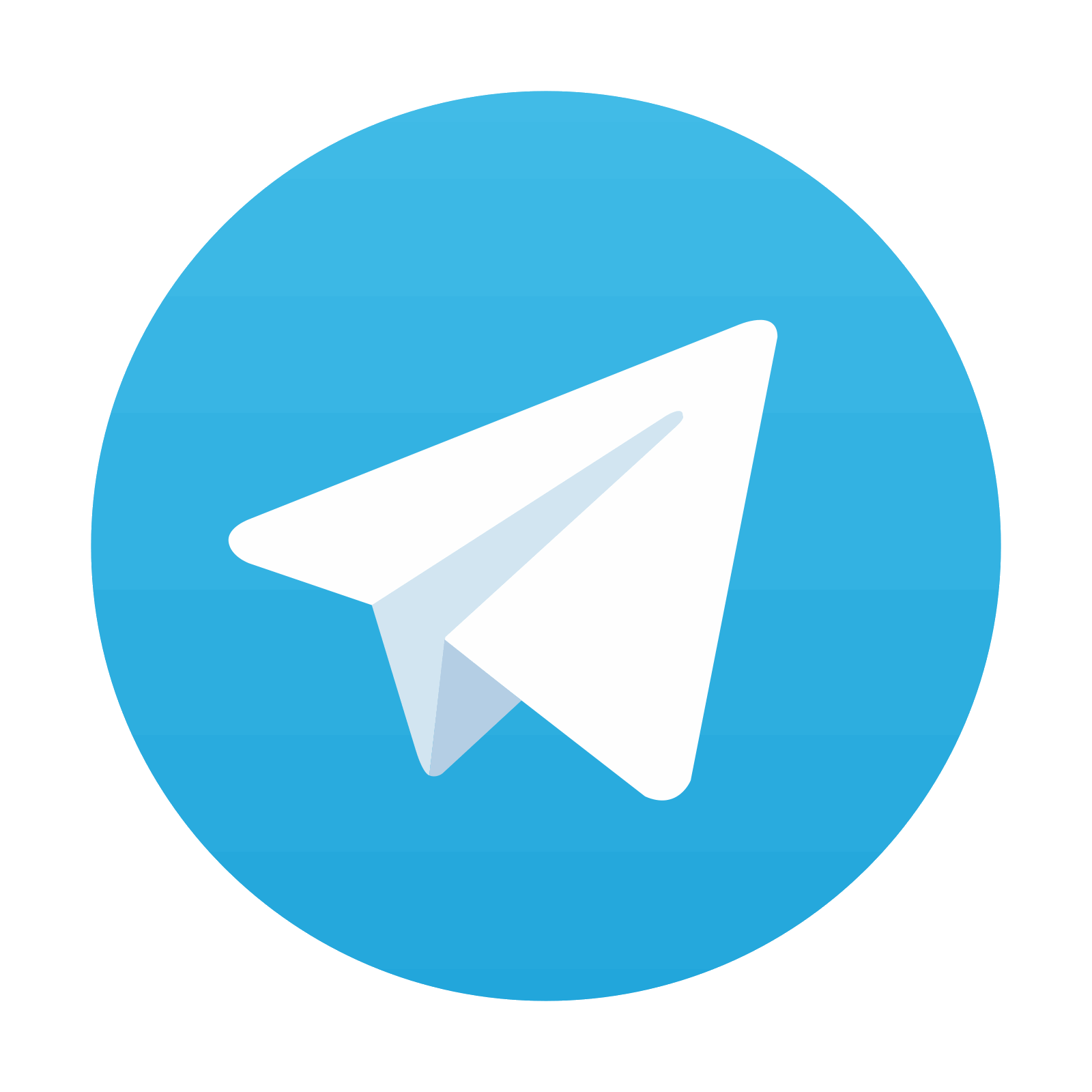
Stay updated, free articles. Join our Telegram channel
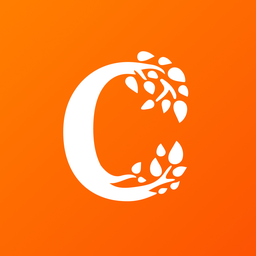
Full access? Get Clinical Tree
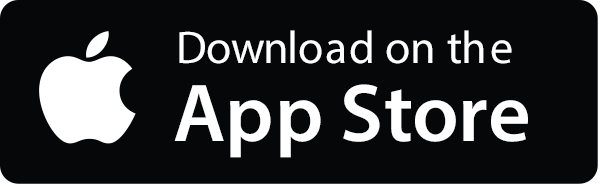
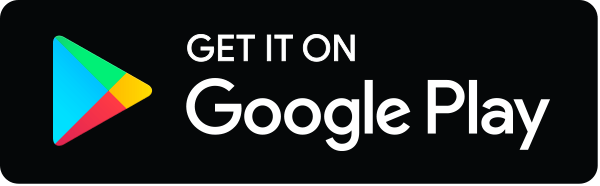