4
Effects of Electrothermal Energy on Cartilage
YAN LU, RYLAND B. EDWARDS, AND MARK D. MARKEL
Articular cartilage lesions, including softening, delamination, and fibrillation, are common findings during arthroscopic evaluation of joints. The management of these disease conditions remains controversial. Some lesions are silent and neither progress nor produce symptoms. Others may produce significant discomfort and progress to severe osteoarthritis and dysfunction. Multiple methods of treatment, including mechanical debridement, microfracture, osteochondral autografts and allografts, chondral autografts, and chondrocyte cell cultures, attempt to provide a more stable and smooth articular surface. Each of these modalities has its drawbacks. Electrothermal energy for chondroplasty is a relatively new treatment option that aims to contour and seal the articular cartilage surface with minimal local damage to chondrocytes and cartilage matrix.
The first time heat (thermal energy) was used in the medical field was by Papyrus in ~3000 BC. In 1514, Giovanni da Vigo stated, “Wounds that are not curable by iron are curable by fire.” In 1540, Johannes Wechtlin began to use a cauterizing “probe” for treatment of patients’ wounds. With the passage of time and technique advancement, electrocautery, laser, and radiofrequency energy (RFE) devices designed for musculoskeletal applications have been increasingly used for the treatment of cartilage lesions.
In 1985, Rand and Gaffey evaluated the effects of electrocautery on fresh human cartilage. Results of this study demonstrated that electrocautery at the lowest setting (27 W) did not cause excessive damage to cartilage. Recently, Stein et al2 determined the clinical effect of electrocautery for arthroscopic chondroplasty of chondromalacic cartilage. This study demonstrated that electrocautery did not produce a significant benefit compared with mechanical chondroplasty alone for the treatment of chondromalacic lesions. Electrocautery appeared to have a deleterious effect on chondromalacic lesions of grade III and higher.
In 1989, Miller et al3 compared the effects of the neodymium:yttrium-aluminum-garnet (Nd:YAG) laser with electrocautery and mechanical debridement on articular cartilage lesions and concluded that lasers had biologic advantages over mechanical resurfacing and electrocautery in arthroscopic procedures. In 1990, Raunest and Lohnert4 reported on the use of the excimer laser for chondromalacic cartilage of Outerbridge grade II and III. The results of this study demonstrated significant pain relief and reduction of reactive synovitis in treated patients. In 1994, Grifka et al5 also reported that the use of excimer laser on chondromalacic cartilage of Outerbridge grade II produced a smooth cartilaginous surface via scanning electron microscopy. Despite these encouraging results, the laser has fallen out of favor in arthroscopic surgery because of high cost, inconvenience, and most importantly, safety concerns about the modality, resulting in osteonecrosis, cartilage loss, and avascular necrosis after laser chondroplasty.6–11 Recently, Lane et al12 and Mainil-Varlet et al13 demonstrated that chondroplasty with laser energy caused significant proteoglycan loss and chondrocyte death.
Before RFE was used for thermal chondroplasty, it was used to correct cardiac electrical conduction pathway abnormalities, to ablate local tumors and cancers, to combat chronic sleep disorders, and to shrink joint capsular tissue and ligaments. It began to be investigated and used for thermal chondroplasty in 1996 to 1997.14 Currently, there are two basic RFE systems available for clinical application: monopolar RFE (mRFE) and bipolar RFE (bRFE). In addition, temperature-controlled RFE probes/generators are available for clinical application with both mRFE and bRFE systems. RFE has the potential advantages of being safe, relatively inexpensive, and simple to use via arthroscopy. It can rapidly contour and smooth fibrillated regions associated with chondromalacic cartilage compared with conventional mechanical debridement, but there are limited in vivo reports regarding its effects on articular cartilage, and the peer-reviewed literature currently provides conflicting results with regard to its safety and efficacy.15,16
Articular Cartilage
Articular cartilage in diarthrodial joints has two primary functions: (1) to distribute joint loads over a wide area, thus decreasing the stresses sustained by the contacting joint surfaces, and (2) to allow relative movement of the opposing joint surfaces with minimal friction and wear. There are three types of cartilage in the human body: hyaline cartilage, fibrocartilage, and elastic cartilage. Healthy or normal hyaline cartilage appears glassy, smooth, and glistening and covers long bones and growth plates; fibrocartilage consists of dense tissue and is located in intervertebral disks and menisci; elastic cartilage is yellowish or opaque and is located in the epiglottis and the eustachian tube.
Cartilage is composed of chondrocytes and matrix. Chondrocytes are sparsely distributed cells in articular cartilage and account for less than 10% of the cartilage’s volume. Chondrocytes also manufacture, secrete, and maintain the organic components of cartilage matrix. The cartilage matrix is composed of a dense network of fine collagen (type II) enmeshed in a concentrated solution of proteoglycans. Collagen and proteoglycans account for 10 to 40% of the matrix, with the remaining 60 to 90% being water, inorganic salts, and small amounts of other matrix proteins, glycoproteins, and lipids. Articular cartilage is unique in that it is devoid of lymphatic, neural, and vascular components. The avascular nature of articular cartilage establishes an environment of low oxygen concentration where chondrocytes rely on anaerobic metabolism.
Chondrocytes are divided into three zones (superficial tangential, middle, and deep) from the cartilage surface to the tidemark (the demarcation between the calcified and noncalcified cartilage). In the superficial tangential zone, chondrocytes are oblong with their long axes aligned parallel to the articular surface. In the middle zone, the chondrocytes are round and randomly distributed. In the deep zone, chondrocytes are arranged in columnar fashion oriented perpendicular to the tidemark. Chondrocytes are the sole cell type in articular cartilage and receive nutrition from synovial fluid flow into the matrix through compression and relaxation during normal joint motion. An important feature of chondrocytes is that they lack direct cell-to-cell contact, and therefore communication between cells must occur via the extracellular matrix. If chondrocytes are injured or damaged, they have very little ability to regenerate.
Collagen in articular cartilage has a high level of structural organization that provides the fibrous ultrastructure to cartilage. Type II collagen is the principal molecular component in cartilage, but collagens III, VI, IX, X, XI, XII, and XIV all contribute to the mature matrix. In developing cartilage, the core fibrillar network is a cross-linked copolymer of collagens II, IX, and XI. The function of collagen IX and XI in this heteropolymer are not yet fully defined, but evidently they are critically important because mutations in collagen IX and XI genes result in chondrodysplasia phenotypes that feature precocious osteoarthritis. Collagens XII and XIV are thought also to be bound to fibril surfaces but not covalently attached. Collagen VI polymerizes into its own type of filamentous network that has multiple adhesion domains for cells and other matrix components. Collagen X is normally restricted to the thin layer of calcified cartilage that interfaces articular cartilage with bone. The collagen in articular cartilage is inhomogeneously distributed, giving the tissue a layered character. Collagens are also divided into three zones (superficial tangential, middle, and deep) within the full thickness of cartilage. In the superficial tangential zone, which represents 10 to 20% of the total thickness, there are sheets of fine, densely packed fibers randomly woven in planes parallel to the articular surface. In the middle zone (40 to 60% of the total thickness), the randomly oriented and homogeneously dispersed fibers are less densely packed to accommodate the high concentration of proteoglycans and water. In the deep zone (~30% of the total thickness), the fibers come together, forming larger, radially oriented fiber bundles; these bundles then cross the tidemark to enter the calcified cartilage, forming an interlocking “root” system that anchors the cartilage to the subchondral bone. This layering inhomogeneity appears to serve an important biomechanical function by distributing the stress more uniformly across the loaded regions of the joint. The most important mechanical properties of collagen fibers are their tensile stiffness and strength.
Cartilage proteoglycans are large protein-polysac-charide molecules that exist as either monomers or aggregates. Proteoglycan monomers consist of an ~200-nm-long protein core to which ~150 glycosaminoglycan chains and both O-linked and N-linked oligosaccharides are covalently attached. In native cartilage, most proteoglycan monomers associate with hyaluronate to form proteoglycan aggregates. Proteoglycan aggregation promotes immobilization of the proteoglycans within the collagen meshwork, adding the structural rigidity to the extracellular matrix. The function of proteoglycans is to resist compression. The proteoglycan and collagen also interact with each other. A small portion of the proteoglycans has been shown to be closely associated with collagen and may serve as a bonding agent between collagen fibrils.
Chondromalacic Cartilage
Chondromalacia is a softening or wearing away and cracking of the articular cartilage. Chondromalacia describes the changes that occur in cartilage covering the ends of bones as it breaks down and degenerates. This condition is not the same as osteoarthritis, and one does not necessarily lead to the other. Osteoarthritis results from long-term wear that causes the lining of the joints to become rough and painful. Chondromalacia results from an injury to otherwise healthy cartilage or as a response to abnormal pressure on the cartilage. An accepted grading scale of chondromalacia by Outerbridge is as follows: grade I, cartilage is softening and swelling; grade II, fibrillation or superficial fissures of cartilage; the fissures in the cartilage are no greater than 1.3 cm2 in area and the fissures do not extend into subchondral bone at this stage; grade III, deep fissuring of the cartilage without exposed subchondral bone; the cartilage has a “crab-meat-like” appearance; and grade IV, erosion changes that extend into subchondral bone and involve more than half of the patellar surface.17 Histologically, chondromalacic cartilage has a fibrillated surface with reduced proteoglycan and collagen staining (Fig. 4–1A). Additionally, chondrocyte clones are found in the middle and deep zones and at the junction of normal and chondromalacic cartilage (Fig. 4–1B).
Because chondromalacia causes discomfort and restricts movement, it usually requires medical evaluation to determine its cause and corresponding treatment. The treatment includes conservative and surgical approaches. Recently, a highly controversial study published by Moseley et al18 demonstrated that conventional treatment for osteoarthritis and chondromalacia using mechanical debridement did not yield a better outcome compared with a placebo procedure.
FIGURE 4–1 (A) Safranin O—stained chondromalacic articular cartilage of grade II or III with surface irregularities, fissures, and reduced proteoglycan concentration (×100). (B) Hematoxylin and eosin (H&E) stained chondromalacic articular cartilage of grade II with surface irregularities, fibrillation, and chondrocyte cloning (&100) (See Color Plate 4–1).
Principles and Techniques of Electrothermal Treatment of Cartilage
The devices used for medical application of thermal energy mainly refer to electrocautery, laser, and radiofrequency modalities. The use of thermal cautery to stop bleeding is an ancient practice. As early as 3000 B.C., tools heated in a fire were used to reduce hemorrhage in accidental injuries. The first units to utilize electrical energy for the heating of cautery instruments date back to the early 1900s. The thermal cautery unit (also called electrocautery) is now a well-established surgical instrument. Electrocautery is a device in which an electric current is used to heat a treatment instrument or probe. When the cautery tip is applied to tissue for coagulation, several changes occur: cells vaporize, removing water from the tissue, causing the tissue to shrink and the blood vessels to contract, or causing the protein from the blood cells and the tissue to form a coagulum. This device does not transfer electric current to the patient. The heat source is the probe tip.
Laser energy is similar in principle to an electro-cautery device, but employs laser energy to cut, vaporize, and simultaneously coagulate a targeted area without disrupting adjacent tissue. “Laser” is an acronym for light amplification by stimulated emission of radiation. When applied to any tissue, light energy can be reflected, transmitted, scattered, or absorbed. In principle, the greater the absorption of light by the target tissue, the more precise and efficient the intended effect. The evolution of laser technology has been based on developing lasers with wavelengths that are optimally absorbed by the musculoskeletal tissue, including the carbon dioxide (CO2), the neodymium, and the holmium lasers. To be clinically useful in sports medicine, lasers must be delivered by reliable fiberoptic technology in an arthroscopic environment. The first clinical studies of laser-assisted arthroscopic surgery were performed with CO2 lasers in the 1970s.19 The main disadvantage of CO2 lasers was that they could not be transmitted through flexible optical fibers. Later, the neodymium:yt-trium-aluminum-garnet (Nd:YAG) laser was used in combination with intraarticularly administered fluid for chondroplasty. The wavelength (1.06 μm) of the Nd:YAG lasers allows both the use of quartz fibers and laser treatment under water. Due to the Nd:YAG’s absorption spectrum, significant collateral thermal damage of the tissue can occur during clinical application of the device, diminishing its usefulness.
Arthroscopic laser systems must cut tissue deeply enough and quickly. The cut edges must be exact, not ragged. Thermal damage and other side effects must be kept to a minimum. There should be no carbonization. The system must be easy to use with a simple laser beam guidance system. These requirements should be met for laser treatment of all tissues, not only for joint cartilage, but also for meniscus, tendons, and even bony tissues. The Nd:YAG laser, therefore, did not fulfill many of these requirements.
Named for the rare element holmium, into which crystals of yttrium, argon, and garnet have been incorporated, the Ho:YAG laser generates a wavelength of 2.1 μm, and fulfills all of the above mentioned requirements, and also has sufficient ablation capabilities to be useful for orthopaedic procedures. Lasers are small in comparison to mechanical instruments and can be used not only in arthroscopic procedures but also for endoscopic procedures. Since the late 1980s, the Ho: YAG laser has been the definitive laser tool used in arthroscopic surgery. Currently, the erbium:YAG (Er:YAG) laser emitting at 2.94 μm appears to have superior effect attributes compared with the Ho:YAG laser for chondroplasty. The Er:YAG laser is known for very shallow optical penetration, in the range of a few micrometers, and for precise ablation with minimal thermal damage to the surrounding tissue.19
The use of RFE for the treatment of fibrillated cartilage has increased tremendously over the past 8 years. The safety and efficacy of this technique is dependent on an understanding of the physics behind RFE. First, RFE is not electrocautery. During RFE application, a high-frequency alternating current flows from the uninsulated probe tip into the tissue. Ionic agitation is produced in the tissue or the irrigation solution about the probe tip as the ions attempt to follow the changes in direction of the alternating current. This agitation results in frictional heating so that the tissue or the irrigation solution about the probe tip, rather than the probe tip itself, is the primary source of heat. The heat produced during RFE application is the difference between the heat generated by RFE current flow through the tissue surrounding the probe tip and the heat lost from this region. The heat generated in the tissue about the probe tip is governed by three factors: (1) distance from the tip; (2) RFE current intensity; and (3) duration of application of the RFE current.
The heat generated in the tissue varies as 1/r4 (where r is the distance between the tissue surface and the probe tip). This implies that tissue-heating changes rapidly with the distance from the probe tip. Physically, a RFE lesion is a prolate spheroid aligned along the electrode tip.
For RFE current intensity, the lesion size generated varies as the intensity is squared. Current intensity, if it is too high and applied too rapidly, will have one of two effects. First, heating of the tissue around the probe tip may be so intense that solidification and char formation will quickly limit further current flow and heating. The lesion size, therefore, will be much smaller than expected. Second, too rapid spread of heat produces scattered areas of explosive water vaporization, resulting in an irregularly shaped lesion larger than the desired size.
Lesion size also depends on the RFE treatment time. For current intensity I, and treatment time T, the RFE heat varies as I2T. Therefore increasing current intensity has a much greater effect on lesion size than increasing treatment time. However, a minimum time is required before a treatment effect will be reached.
Heat loss is a very important factor during RFE application. RFE heat is lost by conduction and by convection via the circulation. At equilibrium, heat generated by the RFE equals heat loss, and lesion temperature remains constant during the remainder of lesion creation. Lesions different in size and shape from those expected result from heat loss by convection in relatively vascularized areas. An indirect form of heat loss can be caused by impaired heat generation through the shunting away of RFE current from a lesion site by the presence of low resistance electrical paths such as cerebrospinal fluid. In this case, the anticipated level of RFE current will be in-adequate because of loss of the heating effect caused by the shunted current.
The size of the RFE probe tip affects lesion size in the expected manner, larger tips producing larger lesions. The effect of increasing the length of the uninsulated tip is a direct result of the 1/r4 attenuation of the heat effect.
Currently, there are three major RFE device systems available for clinical arthroscopic application in thermal chondroplasty. These include the Vulcan EAS™ coupled with a TAC-C probe (Smith & Nephew Endoscopy, Menlo Park, CA), Mitek VAPR™ System coupled with a Flexible Side Effect Electrode (Mitek Surgical Products, Inc., Westwood, MA), and ArthroCare 2000 System™ coupled with CoVac probe (ArthroCare Corp., Sunnyvale, CA) (Fig. 4–2). The Smith & Nephew device is mRFE, whereas the latter two are bRFE devices.
Electrosurgical RFE devices usually use frequencies between 300 kHz and 13 MHz. RFE oscillates the electrolytes in the intracellular and extracellular solutions, producing molecular friction. RFE devices are selected to act at greater than 300 kHz to avoid muscle fasciculation and nerve stimulation observed at lower frequencies.20 During mRFE heating, the alternating electric current passes from the RFE generator through the connecting cable, through the probe (positive electrode), through the patient’s body to the negative electrode (grounding plate).
The mRFE system currently used in clinical application (Vulcan EAS™ RFE generator) is a temperature-controlled device. The mRFE system uses delivered power to control the tissue temperature reflected by a thermocouple within the mRFE probe tip. At the beginning of treatment, the RFE generator delivers full preset power to cause tissue heating. The thermocouple within the mRFE probe tip is subsequently heated, reaching the preset temperature relatively quickly. After reaching the preset temperature, the mRFE algorithm reduces the power to prevent tissue/probe-tip temperature from continuing to increase and then uses bursts of power output to maintain the tissue temperature near the preset temperature. This may result in the mRFE generator delivering mean powers that are significantly less than preset powers to maintain the preset temperatures. The current mRFE (Smith & Nephew, Endoscopy, Menlo Park, CA) setting for chondroplasty is 70°C/15 W. The mRFE chondroplasty should be applied in light contact using a paintbrush pattern.
In the bRFE heating, the alternating electric current passes from the RFE generator through the connecting cable, through the probe, through the positive electrode to the negative electrode, where both positive and negative electrodes are in the probe tip. The conduction pass of the bRFE is within the irrigation fluid, resulting in vaporization of the physiologic saline in the joint. Therefore, the tissue effects with bRFE are secondary to thermal and ionic modification of the tissue.
FIGURE 4–2 (A) Smith & Nephew, Endoscopy, Vulcan EAS™ monopolar radiofrequency energy (mRFE) system. (B) Mitek VAPR™ bipolar RFE (bRFE) system. (C) ArthroCare 2000™ bRFE system.
Most bRFE systems such as Mitek VAPR™ System (Mitek Surgical Products, Inc.) and ArthroCare 2000 System™ (ArthroCare Corp.) are power-controlled devices. These devices produce uniform and direct power output or a variable amplitude sinusoidal waveform while the bRFE probes are activated. No thermocouples are embedded in the probe tips to monitor and adjust the temperature at the interface between the probe tip and treated tissue. Recently, the manufacturer of Mitek introduced a new bRFE (VAPR II Electrosurgical System and VAPR TC Electrode) with temperature-controlled mode for thermal chondroplasty. Shellock21 reported that this new bRFE maintained the temperature at the RFE electrode—tissue interface relatively close to the RFE preset temperature and may be useful for thermal-assisted chondroplasty. The manufacturer of the ArthroCare RFE device also claims an added benefit for thermal chondroplasty using a process they call coblation (cooler, controlled ablation), which the company claims is a non-heat-driven process. Instead, bRFE is applied to a conductive medium (usually saline), causing a highly focused plasma field to form around the electrodes. The plasma field is composed of highly ionized particles. These ionized particles have sufficient energy to break organic molecular bonds within the tissue. Currently, the setting for Mitek bRFE system is V40 and the setting for ArthroCare is setting 2. The bRFE devices should be applied in noncontact using a paintbrush pattern.
Electrothermal Effects on Articular Cartilage
Electrothermal energy devices such as electrocautery, lasers, and RFE have been used to ablate and contour roughened or irregular cartilaginous surface since the 1980s. The results of both experimental and clinical studies have been variable and contradictory.14–16,22
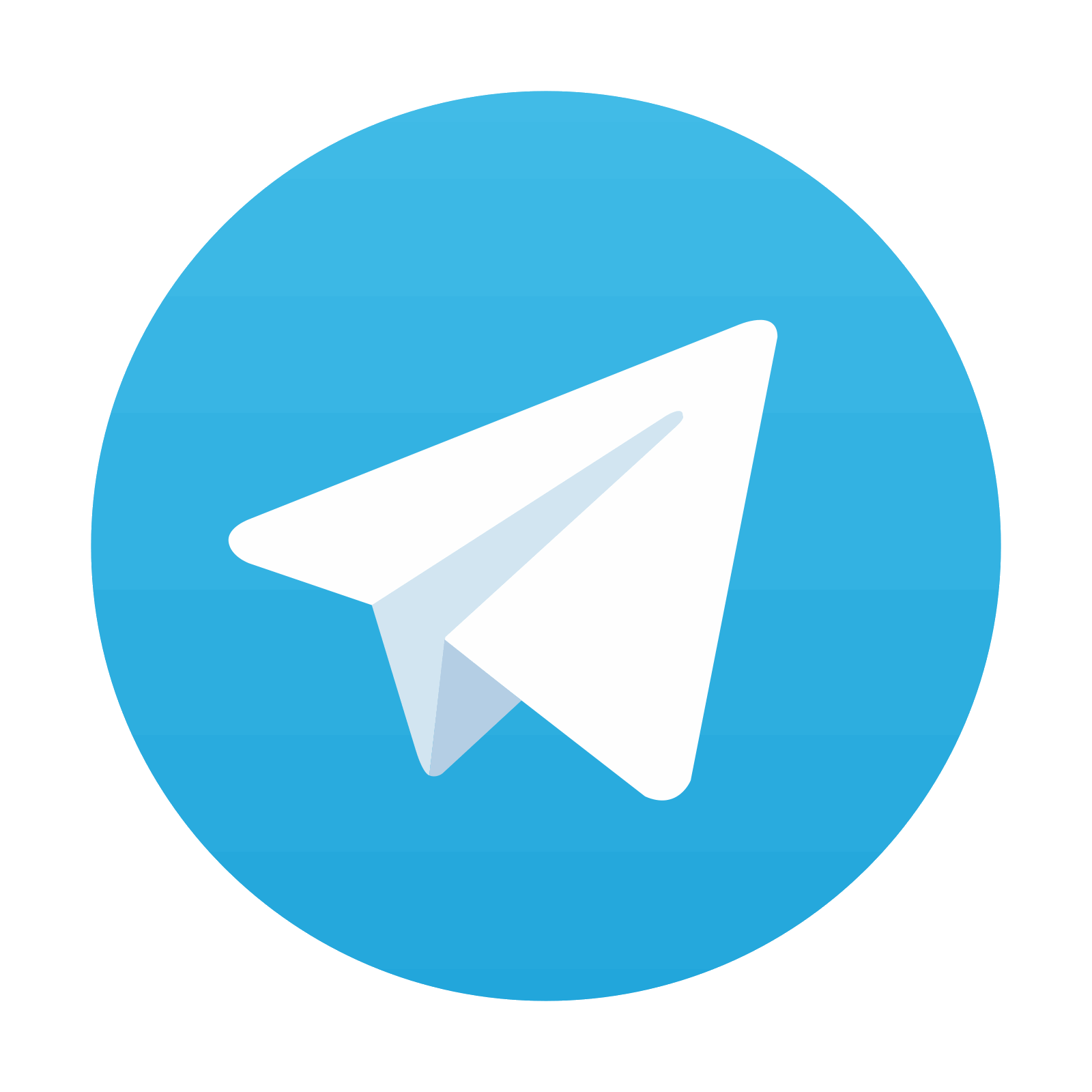
Stay updated, free articles. Join our Telegram channel
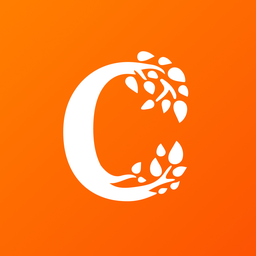
Full access? Get Clinical Tree
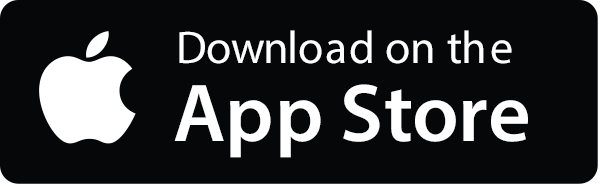
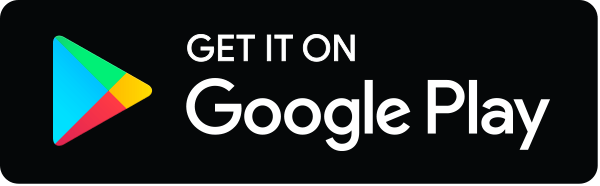