Douglas J. Digirolamo, Emily L. Germain-Lee and Jay Shapiro Bone is a tissue that gives form to the body, supporting its weight, protecting organs and facilitating movement by providing attachments for muscles so that they can act as levers in the musculoskeletal system. Bone also plays a major role in mineral homeostasis, serving as a reservoir for calcium to allow for its precisely controlled levels, critical to proper nerve and muscle function. In addition to these classically defined roles of the skeleton, recent research suggests the skeleton may play an unanticipated role in other aspects of metabolism as well, namely, glucose homeostasis (DiGirolamo et al., 2012). The skeleton consists of a mineralized organic matrix in which highly specialized cells, including osteoblasts (bone-forming cells), osteoclasts (bone-resorbing cells) and osteocytes (a terminally differentiated subset of osteoblasts that reside in the bone matrix) produce and maintain this mineralized matrix through their concerted actions (Marks & Popoff, 1988). Although the basic cellular processes involved in the modeling and remodeling of the skeleton remain the same throughout life, their relative activity – and ultimately skeletal strength and shape – can be influenced by a variety of factors, including hormones, physical activity, pharmacologic agents and nutrition, which guide both the growth and involution of the skeleton with age. Bones vary widely in their shape but can be broadly divided into two categories: flat bones (skull bones, scapula, mandible etc.) and long bones (tibia, femur, humerus etc.). These two categories are based largely upon the method of bone formation by which these bones develop. The bones of the appendicular skeleton, as well as the bones of the vertebral column and base of the skull, are formed by endochondral ossification. In this process, bone forms through a cartilage intermediate that is produced by chondrocytes, providing a template upon which osteoblasts can form new bone. By contrast, flat bones, including most of the bones of the face, the vault of the skull and the pelvis, are formed by intramembranous ossification. In intramembranous ossification, osteoblasts form bone matrix de novo, without a cartilage anlage (Olsen et al., 2000). These two methods of bone formation are present, not only during development, but in adult life as well. For example, a fracture heals via endochondral bone formation, where a cartilage callus is first formed, followed by bone formation in the callus, and finally, remodeling to repair the fracture site to its original anatomic state. Intramembranous bone formation is less common in adult life, but it can be observed in such clinical settings as distraction osteogenesis for limb lengthening. In this procedure, an osteotomy is performed on a long bone and the two ends of bone are separated by a defined distance and externally fixed. This distance is then increased at regular intervals by turning a screw on the external fixator, stimulating bone formation between the bone ends and, thus, lengthening the limb. In the space between the two cut bone ends, known as the distraction gap, osteoblasts form new bone directly, without a cartilage template. Gross inspection of the skeleton reveals that there are two types of bone tissue found in all bones: cortical (compact) bone and trabecular (cancellous) bone. Cortical and trabecular bone have the same matrix composition (predominantly collagen with hydroxyapatite mineral), but the mass of the cortical bone matrix per unit volume is considerably greater due to higher mineral content. Much of the difference between cortical and trabecular bone is secondary to the dual function of bone as both a structural element and a reservoir for calcium in the body. Cortical bone forms the dense outer shell (cortex) of long bones, such as the femur, and bears the majority of mechanical force exerted on the skeleton. This dense (generally 90% by volume calcified) cortical tissue is the predominant type of bone in the diaphysis (midshaft) of long bones, where little or no trabecular bone is present. Moving toward the end of the femur, the thick cortical walls of the diaphysis become thinner and increase in diameter. This region, the metaphysis, is where spicules of trabecular bone emerge, formed from the cartilage template produced by chondrocytes in the epiphyseal growth plate. Still further toward the end of the femur and nearing the knee, atop the epiphysis, is a thin shell of subchondral bone that underlies articular cartilage. As well as occupying the ends of long bones as described above, trabecular bone forms the greater part of each vertebral body and is present at other sites such as the iliac crest. Interestingly, despite accounting for nearly 60% of the surface area of bone in the body, trabecular bone represents only 25% of the total skeletal mass (Fernandez-Tresguerres-Hernandez-Gil et al., 2006). This allows a large surface area for osteoclasts to resorb bone mineral and release calcium rapidly when necessary, while the dense cortical bone remains relatively unaffected and capable of bearing the mechanical loads applied to the skeleton. Trabecular bone is the most metabolically active compartment of the skeleton, with a high rate of turnover and a blood supply that is much greater than that of cortical bone (Banse, 2002). To carry out the diverse functions of bone formation and bone resorption, as well as maintain mineral homeostasis, bone cells assume specialized forms categorized by function, morphology and characteristic location. Bone cells derive from two distinct progenitor cell types: mesenchymal stem/stromal cells (MSCs) and hematopoietic stem cells (HSCs). The mesenchymal cells give rise to bone-lining cells, pre-osteoblasts, osteoblasts and osteocytes. Hematopoietic stem cells, which are also residents of the bone marrow and reside within specialized ‘niches’, give rise to monocyte/macrophage precursors (as well as many other immune cell lineages not immediately relevant to the discussion of bone cells) that ultimately differentiate into pre-osteoclasts and osteoclasts. Undifferentiated MSCs that have the potential to become osteoblasts reside in bone canals, along the endosteum (inner lining of the cortex), periosteum (outer lining of the cortex), trabeculae and within the marrow. These cells, under the control of numerous growth factors and hormones – like sex steroids, growth hormone (GH) and insulin-like growth factor-1 (IGF-1) – will undergo proliferation, differentiate into pre-osteoblasts, and finally become mature osteoblasts to form new bone. Mature, bone-forming osteoblasts never appear or function individually but are always found in clusters along the bone surface where they produce osteoid – bone matrix composed predominantly of highly ordered collagen fibrils, upon which hydroxyapatite mineral forms. Upon completing bone formation, active osteoblasts follow one of three courses: they may remain on the surface of the bone, decrease their synthetic activity and assume the flatter form of bone-lining cells; they may surround themselves with matrix, further differentiate to become osteocytes and continue mineralizing bone (roughly 10–20%); or they may undergo programmed cell death and disappear from the site of bone formation. Osteoclasts are large, multinucleated cells found on bone surfaces that are responsible for bone resorption. Specific hormones and growth factors produced by osteoblasts and osteocytes (as well as other cell types in pathological conditions) influence their development from monocyte/macrophage precursors. These precursors differentiate and fuse together to form osteoclasts on the surface of bone, where they are very efficient at destroying bone matrix. They begin by binding to the surface of the bone and creating a sealed space between the cell membrane and the bone matrix. Osteoclasts then use membrane bound proton pumps to transport protons into, and acidify, the sealed space, decreasing the pH from ~7 to 4. This acidic environment solubilizes the hydroxyapatite mineral found in bone, releases calcium and phosphate, and allows access to the organic matrix, which is degraded by osteoclast-secreted acid proteases. Growth factors bound in the organic matrix are also released during this process, which stimulates osteoblast formation and, thus, couples the two processes to ensure proper maintenance of bone mass (Fernandez-Tresguerres-Hernandez-Gil et al., 2006). Osteocytes, as mentioned previously, differentiate from a subset of mature osteoblasts and become entombed within lacunae in the newly formed, mineralizing bone matrix. During this process, they form an extensive network of neuron-like cell projections, connecting them to other osteocytes, osteoblasts, bone-lining cells, blood vessels and nerves through a vast canalicular network in bone. Unlike the relatively short-lived osteoclasts (~3 weeks) and osteoblasts (~3 months), osteocytes have an estimated life span of 10–20 years in humans and comprise 90–95% of bone cells. Although their precise function has yet to be clearly established, osteocytes are widely assumed to play a role in transducing mechanical forces into anabolic signals within bone, allowing bone to adapt to increased or decreased activity. More recently, they have been demonstrated to produce factors that can regulate both osteoblast and osteoclast development, implicating them as a possible ‘conductor’ in orchestrating bone remodeling. In addition, osteocytes secrete a growth factor known as fibroblast growth factor 23 (FGF23), which regulates phosphate homeostasis through endocrine actions in the kidney (Bonewald, 2011). Despite their populous nature and obvious importance in bone physiology, many aspects of osteocyte function remain undefined due to their highly specialized microenvironment in bone and the inherent technical difficulty in generating faithful model systems. Throughout life, physiological remodeling (removal and replacement) of bone occurs without affecting the shape or density of the bone. In fact, bone is far more dynamic than many people appreciate, with the entire human skeleton being replaced through the process of normal remodeling approximately every 10 years. A complete remodeling cycle includes osteoclast activation, resorption of bone, osteoblast activation, formation of new bone at the site of resorption and reversion to a resting state. Skeletal remodeling takes place in response to a number of growth factors, hormones and mechanical signals, some of which have already been discussed. This continual remodeling serves to repair micro-damage that accumulates in the bone over time (or major damage in the case of fracture) and ensures the mechanical integrity of bone (Cardoso et al., 2009; Herman et al., 2010), as well as maintains calcium and phosphate homeostasis (Quarles, 2008). It should be noted that this process is distinct from bone modeling, in which osteoblasts form bone continuously without a resorptive cycle. One such example of modeling is periosteal apposition during pubertal growth, in which periosteal osteoblasts continuously deposit new bone on the periosteal surface to increase the cross-sectional area of long bones, dramatically increasing their strength (Bachrach & Asbmr, 2009). Remodeling occurs both on the surface of trabecular and cortical bone, as well as within cortical bone. Internal, or osteonal, remodeling begins when osteoclasts create a tunnel through bone. These cutting cones of osteoclasts create large resorption cavities. Immediately behind the cutting cones, groups of osteoblasts follow the advancing osteoclasts. Layers of osteoblasts arrange themselves along the surface of the resorption cavity behind the osteoclast and deposit successive lamellae of new bone matrix, which then mineralize and fill the canal. In normal adult bone, remodeling is usually a tightly controlled process in which bone resorption equals bone formation, achieved through teams of osteoblasts and osteoclasts forming tightly regulated basic multicellular units (BMUs) or bone remodeling units (BRUs) (Dempster, 2003; Kular et al., 2012). Unfortunately, this delicate balance inherently favors the destruction of bone, as the resorptive portion of a remodeling cycle takes ~3 weeks, compared to the ~3 months required for complete bone formation. The nature of this inherent imbalance between formation and resorption time can be easily appreciated if one imagines a packet of bone as a moving truck. Unpacking a moving truck (resorption) is relatively quick and easy, as one simply has to pull out the contents and put them elsewhere. By contrast, packing a moving truck (formation) requires careful attention to packing the items into boxes, carefully arranging those boxes to fit into the cargo space and then securing them all for the move (in the case of our metaphor – producing collagen, secreting and aligning collagen fibrils, and mineralizing the newly formed matrix). Primary osteoporosis is defined as a pathological unbalancing of bone resorption and formation that leads to persistent deficits of bone mass, which ultimately translates into increased fracture susceptibility. Changes in bone homeostasis may also result secondary to a number of other pathological conditions (e.g. cancer, kidney disease, endocrine disorders etc.). The peak bone mass attained in adult life is governed by a combination of genetic, hormonal (predominantly estrogen, GH and IGF-1), nutritional and mechanical factors (Bonjour et al., 1994). In humans, this peak bone mass is attained in the third decade of life and is a large determinant of one’s susceptibility to fractures later in life regardless of sex, race etc. This is because a slow loss of bone mass (~0.5% per year) begins around age 40 in both sexes and continues for the rest of life (Mazess, 1982; Clarke & Khosla, 2010). Thus, the greater the peak bone mass, the longer it will take to reach the threshold of bone density at which fracture risk begins to rise. At all ages, however, women have a lower bone mass than men and, with increasing age, this gap widens. This widening gap is largely the result of an accelerated period of bone loss in women around the time of menopause, when bone loss rates of 5–6% per year for up to 10 years are not unusual. This accelerated loss is associated with the withdrawal of estrogen (Dempster, 2003; Downey & Siegel, 2006; Clarke & Khosla, 2010), which results in a dramatic increase in bone resorption – as much as 90% as indicated by biochemical markers. Bone formation markers are also increased during menopause (although formation decreases later), but only by 45%, thus favoring the high turnover bone loss that decimates bone density in postmenopausal women. Men do not suffer the sudden drop in sex steroid levels and concomitant rapid bone loss observed in women, and they are, therefore, less susceptible to fracture than females with age because they retain a greater percentage of their bone mass. The slow bone loss that is observed in men was long assumed to result from reduced serum testosterone, as it predominates in many other aspects of male physiology. In recent years, however, numerous studies have proved this not to be the case. In fact, reduced estrogen – converted by aromatase from testosterone – is responsible for age-related bone loss in men. Further, estrogen actually appears to be critical for proper bone growth in men earlier in life. Thus, throughout life in both genders (even into the eighth and ninth decades), estrogen has significant anabolic effects on bone, and its reduction accounts for a large portion of age-related bone loss (Raisz, 2005; Clarke & Khosla, 2010). In both genders, levels of parathyroid hormone (PTH) tend to gradually increase with age (Ledger et al., 1995). Parathyroid hormone is responsible for maintaining calcium balance in the body, in concert with vitamin D, by regulating bone resorption, calcium reabsorption in the kidney and calcium absorption in the small intestine (Quarles, 2008). Although this gradual rise in PTH level is certainly multifactorial, vitamin D deficiency is common among the elderly and likely contributes to bone loss by disturbing this PTH–vitamin D endocrine loop. Thus, as blood calcium would drop from reduced vitamin D (thereby less intestinal calcium absorption and renal reabsorption of calcium), PTH would stimulate additional bone resorption to maintain healthy blood calcium levels, thereby further contributing to age-related bone loss. Although the cause of vitamin D deficiency with age remains unclear, recent studies have identified an inverse correlation between BMI and vitamin D levels (Wortsman et al., 2000; Vimaleswaran et al., 2013). It has been suggested that the reduction in vitamin D may result from the increased fat mass capable of storing vitamin D and thus sequestering it from the circulation, providing a possible explanation for reduced vitamin D levels in the elderly since body fat, much to our chagrin, unavoidably increases with age. As mentioned previously, bone formation rates decline in both sexes with increasing age. This drop in osteoblast function and bone formation parallels the reduction in GH and IGF-1 levels observed in aging (Perrini et al., 2010). These two factors have been demonstrated, in numerous studies, to be critical for osteoblast proliferation, survival and function, particularly mineralization. The GH/IGF-1 axis is also intimately linked to the positive effects exerted by sex steroids on bone, particularly during pubertal growth (Olson et al., 2011). Thus, it is likely that the decline of GH and IGF-1 levels also contributes significantly to age-related bone loss by failing to sufficiently stimulate bone formation. Immobility (Fox et al., 2000), systemic acidosis (as might be seen with poor kidney function) (Arnett, 2003) and some pharmaceutical agents (e.g. glucocorticoids and corticosteroids) (Weinstein, 2012) can also impact on bone mass in a negative fashion. Unfortunately, it is not uncommon for an individual to encounter one or more of these negative modifiers with increasing age. In this regard, reduced activity and/or immobility due to injury pose an especially significant risk for exacerbating frailty and increasing fracture risk in an elderly population. Muscle mass and function also suffer significant loss with disuse and aging (Jang & Van Remmen, 2011; Marimuthu et al., 2011), leading to an increased propensity for falls and, with concomitant bone loss, even greater risk of fracture. Other factors that can impair osteoblast function and stimulate osteoclast formation/activity include chronic inflammation – possibly associated with cellular senescence (Freund et al., 2010) – and accumulated oxidative damage (Almeida et al., 2007), as might be observed with long-term obesity. Finally, nutrition also contributes to bone loss with age, beyond simply vitamin D and calcium intake, since appetite decreases. This decrease in appetite results in a reduction of both macro- and micronutrients across the board. For example, reduced protein intake has been linked to poorer bone mass in the elderly (Bonjour, 2011). This observation is not surprising when one considers that the vast majority of bone is organic collagen matrix, which requires adequate protein building blocks to produce and maintain. Recent advances in our understanding of the molecular signals regulating bone and muscle growth have identified new strategies to enhance bone mass, either directly or indirectly. Of course, the most effective strategy to prevent bone loss and reduce fracture risk begins much earlier in life by attaining the highest peak bone mass possible and raising the ‘starting point’ for inevitable bone decline. Maintaining high activity levels with age is also critical to help reduce the rate at which bone mass declines as a result of the many factors described above. Higher activity levels with age also help to avoid frailty by maintaining greater muscle mass and function, which helps to maintain balance and coordination. Unfortunately, these proactive measures may not be sufficient if genetics happen not to be on one’s side in the fight against bone loss. In such a case, a number of pharmaceutical interventions are also available to help stave off age-related bone loss. Bisphosphonates are the current standard therapy for bone loss and help spare bone mass by blocking osteoclasts from resorbing bone, although they cannot replace lost bone mass. By contrast, intermittent administration of PTH can replace lost bone mass (and is currently the only pharmaceutical available that is capable of this action), although the mechanism by which it does so remains elusive. Novel biologics are emerging as well, which exploit our knowledge of the bone remodeling pathways to help tip the balance back in favor of formation. One example of such a biologic is denosumab (marketed under the trade name Prolia), which is an antibody that binds and inhibits a factor that promotes osteoclast formation, known as RANKL. In addition to such strategies that directly target aspects of the bone remodeling process, recent studies have demonstrated a viable alternative to enhance bone mass indirectly by increasing the mechanical load on bone, via increased muscle. One example of such a strategy is to increase muscle mass by targeting pathways that would normally suppress muscle growth, such as myostatin (McPherron et al., 1997). Myostatin is produced by skeletal muscle cells, circulates in the blood and acts to limit muscle cell hypertrophy. When the activity of myostatin is lost by naturally occurring mutations in the gene, this suppressive force is relieved and muscles attain striking size and mass. Perhaps not surprisingly, given the known effects of muscle force to promote maintenance or increase of bone mass, animals with myostatin mutations also have markedly increased bone mineral density (Hamrick et al., 2003). Although the precise mechanisms by which myostatin is normally regulated and exerts these effects in muscle are still being elucidated, its activity can be blocked artificially by a variety of both naturally occurring and engineered myostatin-binding proteins (reviewed in Lee, 2004, 2012). It is not hard to envision how beneficial such a therapy could be in addressing age-related frailty, as the improved muscle mass would not only help to improve bone mass indirectly, but also improve balance, coordination and mobility to further reduce risk of falls and fractures. The rapid increase in the understanding of the mechanisms that control the development and regulation of the musculoskeletal system has led to many advances in our approach to treating age-related bone loss. The ability to manipulate formation and resorption of bone, both directly and indirectly, will substantially improve the treatment of musculoskeletal disorders. Further, interventions that exploit this knowledge of bone cell function offer the potential to treat numerous other diseases with skeletal involvement.
Effects of aging on bone
Introduction
Bone structure
Macroscopic anatomy
Microscopic anatomy
Bone remodeling
Age-Related changes in bone
Sex steroids
Secondary hyperparathyroidism
GH/IGF-1 axis
Other factors
Future directions
Conclusion
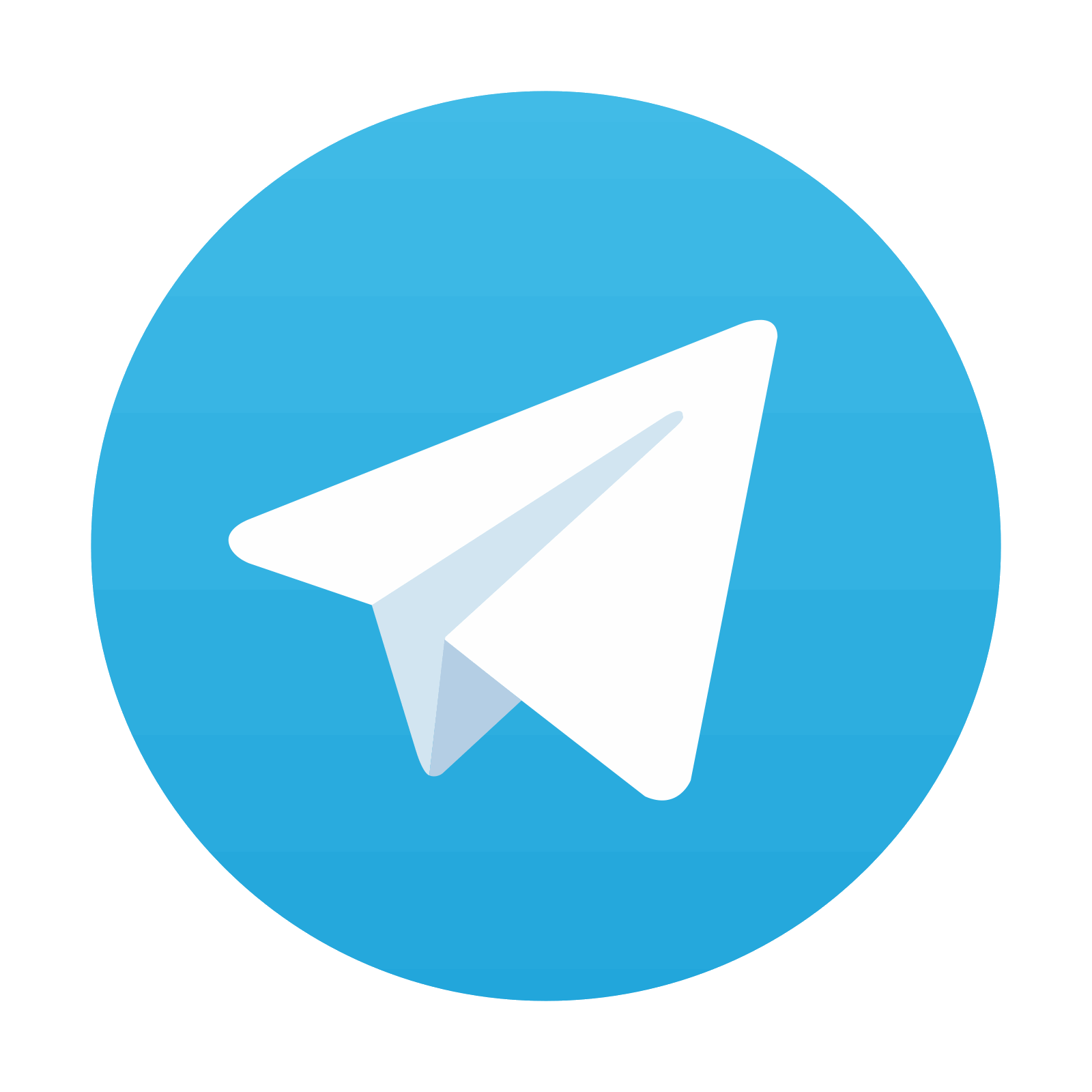