Fig. 9.1
The main roles of the PCr–Cr system are illustrated in this figure. The first is that of a temporal energy buffer for ATP regeneration achieved via anaerobic degradation of PCr to Cr and rephosphorylation of ADP. This energy buffering function is most prominent in the fast-twitch/glycolytic fibers; these fibers contain the largest pool size of PCr [6]. The ATP required for high-intensity exercise is met by the simultaneous breakdown of PCr and anaerobic glycolysis of which the PCr–Cr system provides up to one-third of the total energy required [7]. The second major function of the PCr–Cr system is that of a spatial energy buffer (or transport system). In this capacity, the PCr–Cr system serves as an intracellular energy carrier connecting sites of energy production (mitochondria) with sites of energy utilization (Na+/K+ pump, myofibrils, and the SR) (see figure). To describe the specificity of this system, it has been coined the creatine-phosphate (Cr-Pi) shuttle [8]. Meaning, Cr literally shuttles energy from the mitochondrion to highly specific sites via compartment-specific creatine kinase (CK) isoenzymes located at each of the energy-producing or energy-utilizing sites that transduce the PCr to ATP [5] and then returns to regenerate energy exactly equivalent to its consumption at those sites [8]. A third function of the PCr–Cr system is the prevention of a rise in ADP that would have an inhibitory effect on a variety of ATP-dependant processes, such as cross-bridge cycling. A rise in ADP production would also activate the kinase reactions that ultimately result in the destruction of muscle adenine nucleotides [2]. Therefore, the removal of ADP via the creatine kinase (CK) reaction-induced rephosphorylation serves to reduce the loss of adenine nucleotides while maintaining a high intracellular ATP/ADP ratio at the sites of high energy requirements [9]
9.2.1 Why Creatine?
If we condense the information covered so far, the ergogenic potential of Cr becomes clear. Cr is a naturally occurring tripeptide found prominently in active muscle [1, 2]. However, under normal circumstances, muscle stores of Cr are not saturated [11]. Whether synthesized endogenously or consumed orally, Cr is phosphorylated and stored within muscle [3, 4]. Therefore supplementation represents a unique opportunity to increase cellular reserves to provide large amounts of energy that enhance the cellular bioenergetics of the PCr–Cr system by increasing PCr availability [2, 9, 12]. While metabolic investigations involving Cr date back to the 1830s, the exogenous use of Cr in published research can be found in the early 1900s [13]. Cr as a supplement used for medical interventions such as treating diabetes and gyrate atrophy is documented from the 1930s up to the 1960s and 1970s [1–3]. However, the earliest scientific investigations involving the deliberate use of Cr to enhance athletic performance belong to Drs Eric Hultman, Karin Soderlund, and Roger Harris. These researchers pioneered the seminal work that showed large oral doses (20–30 g daily) of Cr in the form of creatine monohydrate (CrM) could increase plasma Cr and accumulation in skeletal muscle [1–3, 11, 12, 14]. Hultman was also one of the pioneers of CHO loading to enhance glycogen supercompensation and performance in the 1960s [15–17]. Hultman and colleagues applied the same loading principles with CrM supplementation [11, 12, 14].
CrM (n[aminoiminomethyl]-N-methylglycine) is a combination of Cr (anhydrous) and one water molecule. CrM is particularly stable even at high temperatures (stored over 60 °C for 3 years) and has slow degradation even at low pH levels [18]. Intestinal absorption of CrM is close to 100 % [19], Commercially, CrM is inexpensive to produce (compared to most supplements) and yields very high Cr purity (over 90 %) [18]. Probably for these reasons CrM supplementation has been the focus of well over 500 published investigations that have established that “loading” (4 × 5-g servings · day−1, 3–5 days) is a safe and effective way to elevate muscle Cr concentrations by 15–40 % [1, 11] and enhance athletic performance under a variety of circumstances [14, 20–25]. Regular use also appears to enhance the chronic adaptations desired from resistance training [26–28]. A large scientific body of literature continues to document this supplement’s physiological [29–32] and performance-enhancing [33–37] effects as well as dispel concerns of adverse effects [27, 38]. For these reasons, there has always been interest in combining CrM with other compounds to enhance its ergogenic potential.
9.2.2 Why Combine Creatine with Other Compounds?
The beneficial effect of oral supplementation is thought to be dependent on the extent of Cr accumulation [12, 26, 28]. However, it is also apparent that this response can be highly variable between subjects [39]. Large variations in Cr accumulation (0–40 mmol · kg dm−1) in response to supplementation can be partly accounted for by differences in pre-supplementation muscle concentrations [28] and possibly in muscle fiber-type distribution [6], but it remains unclear as to why muscle Cr accumulation can vary tremendously (up to sixfold) among individuals with similar pre-supplementation concentrations [21, 39, 40]. This variability in muscle Cr uptake among some individuals combined with the significance of the PCr–Cr system and CrM’s potential to augment this all-important pathway is the underlining rational of studies that examine the effects of CrM supplementation in combination with other compounds.
9.2.3 The Effects of Other Compounds on Creatine Uptake
The presence of phosphatase enzymes in the blood and gut suggests that supplementation with other energy-yielding components of the phosphagen system, such as ATP or PCr, is not a viable option as these enzymes readily cleave the phosphate from the molecule [41]. Whereas the Cr portion of the monohydrate form consists of up to 92 % Cr, it only forms 50 % of the PCr molecule [42]. Oral supplementation with CrM enters the circulation intact where active uptake by tissues is facilitated by a Na+-dependent transporter against a concentration gradient [43]. CrM’s capacity to enhance the bioenergetics of the phosphagen system by increasing PCr availability is thought to reside in the extent of Cr accumulation within muscle [12, 26, 28]. To exert a beneficial effect on performance and metabolism, an increase in muscle total Cr (PCr + Cr) content by at least 20 mmol · kg dm−1 appears to be required [40]. While a loading phase is shown consistently to achieve this (as well as increase total Cr concentrations in other tissues with low baseline Cr content), it is also apparent that this response can be highly variable between subjects. For these reasons, a number of studies have assessed Cr uptake in the presence of other compounds. For example, Cr accumulation in muscle is enhanced by the presence of insulin [44] and possibly triiodothyronine [45] but may be depressed by the presence of some drugs such as ouabain or digoxin [46] or a vitamin E deficiency [47]. The findings from some investigations suggest that caffeine may impair the advantages of Cr loading [48], whereas other studies have involved caffeine-containing beverages (such as tea or coffee) to administer CrM and report significant elevations in muscle Cr as well as improvements in athletic performance [7, 9, 14, 21]. Other investigations report that muscle Cr uptake is not affected by PCr, creatinine, or cellular concentrations of various amino acids such as glycine, glutamine, alanine, arginine, leucine, and glycine or the sulfur-containing amino acids methionine and cysteine [49, 50].
9.2.4 Creatine with Macronutrients
Improved cellular retention of Cr has been attributed to a stimulatory effect of insulin on the Cr transporter protein [44]. Carbohydrates (CHO), such as glucose and sucrose, generally evoke a high insulin response. Once it had been demonstrated that the presence of insulin (at supraphysiological levels) increased muscle Cr accumulation in humans [40], other investigations that examined the effects of combining CrM with CHO such as glucose soon followed. In two separate studies, Green et al. were the first to demonstrate reduced urine Cr losses [51] and a 60 % increase in muscle Cr accumulation [52] from combining a high dose of glucose (93 g) with each 5 g dose of CrM (4 × 5 g · day−1, 2 days) compared to CrM alone. Robinson et al. [53] also showed that a high-CHO diet combined with CrM (4 × 5 g · day−1, 5 days) after exercise provided effective (P < 0.01) Cr accumulation in the exercised limb. However, data from subsequent studies suggested that lower doses of CHO (glucose) may also be effective. For instance, Greenwood et al. [54] assessed whole-body Cr retention (via 24-h urine samples for 4 days) and reported that a 5-g dose of CrM combined with an 18-g dose of glucose (4× · day−1, 3 days) resulted in significantly greater Cr retention than an equivalent dose of CrM only, or an effervescent Cr supplement (containing sodium and potassium bicarbonate). Along this line, Preen et al. [55] examined the effectiveness of 3 different CrM-loading procedures on total Cr accumulation in muscle. Eighteen physically active males were divided into three equal groups and provided either (a) CrM (4 × 5 g · day−1, 5 days), (b) the same dose of CrM + glucose (1 g · kg−1, twice · day−1, 5 days), or (c) CrM combined with 60 min of daily exercise (repeated sprints) (CrM + E) for 5 days. Results showed that the combination of CrM + glucose provided a 7–9 % greater (P < 0.05) elevation in total muscle Cr concentrations than CrM only or CrM + E [55].
Supplementation with high insulin-stimulating CHO appears to be effective at promoting Cr uptake; however, combining CrM with a protein (PRO) supplement may also provide similar benefits. For example, using a group of recreational weight lifters, one study directly compared the effects of two CrM-containing supplements, CrM + CHO (glucose) and CrM + PRO (whey isolate) (1.5 g of supplement · kg · day−1) during 11 weeks of resistance training [56]. After the 11-week program, the two different CrM-containing supplements provided a similar increase in total muscle Cr concentrations (~10 %). Additionally, the groups given the CrM + CHO and CrM + PRO supplements demonstrated greater (P < 0.05) strength improvements and muscle hypertrophy compared to an equivalent dose of CHO or PRO [56]. Other studies have reported similar benefits from combining CrM with whey PRO [57] or CHO (glucose) [24] during resistance training, but muscle Cr concentrations were not assessed. Whereas these studies utilized relatively large doses of PRO or CHO (70–100 g or more) in combination with CrM [56–58] and reported positive outcomes, the results of one study by Stout et al. [59] suggest that a smaller dose of CHO (35 g glucose) with each 5-g dose of CrM is also effective at improving training adaptations. However, no other studies have directly compared the effects of different CrM-containing PRO or CHO supplements on Cr accumulation and training adaptations.
Combining PRO, CHO, and CrM may be the most effective mix for promoting whole-body Cr accumulation, particularly if smaller doses of the macronutrients are desired. Steenge et al. [40] reported that the ingestion of CrM along with a PRO + CHO supplement (50 g dairy milk PRO, 50 g glucose) over 5 days resulted in similar insulin responses and (whole-body) percentage Cr accumulation values (~25 %) as the same CrM dose combined with 100 g of glucose [40]. Whole-body Cr accumulation is an indirect method assessing CrM uptake by tissues. Percent whole-body Cr retention can be calculated as Cr ingested (g)/urinary Cr excretion (g) × 100 [40]. The results obtained by Steenge et al. [40] suggest that the combination of PRO and CHO with CrM may be an effective way to improve Cr accumulation particularly when smaller doses of these macronutrients are desired. This combination may also have important implications for populations where the consumption of large amounts of CHO is undesirable, such as those with, or at risk of, type-II diabetes. This combination (CHO–PRO–CrM) has also been used to demonstrate that the timing of supplementation may also be important to improving Cr accumulation in muscle and adaptations from training [60]. Other studies have shown that CrM supplementation close to exercise promotes muscle Cr uptake [53] and increases the girth and thickness of the exercised limb after resistance training [61]. Therefore, the use of a CrM-containing PRO–CHO supplement before and after resistance exercise may provide a higher degree of Cr accumulation and muscle anabolism and, therefore, promote better gains in strength and muscle mass. To summarize the research in this particular area, co-ingestion of CrM with CHO and/or PRO (namely, glucose or whey isolates, ~35–100 g) appears to enhance muscle Cr storage that may result in enhanced performance and better training adaptations. Greater accumulation within muscle appears to be due to a stimulatory effect of insulin on cell Cr transporter. In fact, combining CrM with a PRO and/or CHO supplement seems to reduce the individual variations in muscle Cr accumulation reported previously in studies involving acute loading [21, 39, 40]. Additionally, there is evidence to suggest that the timing of the supplement dose is important. The use of this supplement combination close to exercise (i.e., just before and/or after) appears to promote better Cr accumulation within muscle and influence training adaptations [53, 60]. Therefore, the use of a CrM-containing PRO–CHO supplement close to exercise represents a simple but highly effective strategy that promotes effective Cr accumulation (to increase PCr availability in muscle) and provides an ergogenic effect during training that results in greater adaptations. Further examination of dose–responses alongside the extent of Cr accumulation and adaptations would help define a clearer supplementation prescription.
9.2.5 Creatine with Insulin Mimickers
Aside from the use of macronutrients such as PRO and CHO, some studies have examined the effects of co-ingesting CrM with other compounds that affect insulin secretion and/or tissue sensitivity. For instance, in a single-blinded study, Greenwood et al. [62] examined whether co-ingestion of d-pinitol (a plant extract with insulin-sensitizing characteristics) [63] with CrM affected whole-body Cr retention (determined by 24-h urine samples for 4 days). Results revealed that whole-body Cr retention (and percentage of Cr retention) over the 3-day loading phase was greater (P < 0.05) in the two groups given CrM combined with a low dose of d-pinitol (LP = 4 × 5 g CrM + 2 × 0.5 g d-pinitol) (PreP = d-pinitol 2 × 0.5 g d-pinitol 5 days prior to and during CrM supplementation 4 × 5 g CrM) compared to equivalent dose of glucose (placebo) or CrM alone. However, another group given a high dose of d-pinitol (4 × 0.5 g d-pinitol) with the same dose of CrM showed no greater Cr retention when compared to the group given CrM alone [62]. Interestingly, the group pre-dosing with d-pinitol (PreP) demonstrated the same results as the LP group suggesting that no further benefit seems to be gained by taking d-pinitol prior to supplementation [62]. In a follow-up study, Kerksick et al. [64] examined whether co-ingestion of d-pinitol with CM would affect training adaptations, body composition, and/or whole-body Cr retention in resistance-trained males. In the study, 24 resistance-trained males were randomly assigned in a double-blind manner to CM + D-pinitol or CM alone prior to beginning a supervised 4-week resistance-training program. Subjects ingested a typical loading phase (i.e., 20 g · day−1 for 5 days) before ingesting 5 g · d−1 for the remaining 23 days. The researchers reported that Cr retention increased in both groups as a result of supplementation. However, no significant differences were observed between groups in training adaptations. The authors concluded that ingesting Cr with d-pinitol may augment whole-body Cr retention in a similar manner to that reported with CHO or CHO and PRO supplementation [40]. Due to the conflicting nature of the results regarding the high vs. low doses of d-pinitol, further research is necessary before a clear conclusion can be drawn.
Another insulin mimicker that has shown potential to enhance Cr uptake and accumulation in muscle is α-lipoic acid (ALA). Supplementation with ALA is shown to increase the expression of glucose transporter proteins (GLUT4) and enhance glucose uptake in muscle [65, 66]. In light of the fact that Cr uptake is influenced by insulin and that ALA can increase glucose disposal, Burke et al. [67] examined the effects of combining ALA with CrM on muscle Cr accumulation. In this study muscle biopsies were obtained to determine total Cr concentration from16 male subjects before and after the 5-day supplementation intervention. Results showed a greater increase (P < 0.05) in PCr and total Cr in the group given ALA combined with CrM + CHO (20 g · day−1 CrM + 100 g · day−1 sucrose + 1,000 mg · day−1 ALA) compared with a group given the same dose of CrM + CHO or CrM alone. The authors concluded that co-ingestion of ALA with CrM (and a small amount of sucrose) can enhance muscle Cr concentrations content compared to an equivalent dose of CrM + CHO or CHO alone [67]. However, the authors also acknowledge that a limitation of this study was the high baseline muscle Cr concentrations exhibited by the participants; the groups were ~10 % higher than starting values reported in other studies (~135 mmol · kg−1 vs. ~125 mmol · kg−1). Initial muscle Cr content is an important determinant of muscle Cr uptake [40]. That is, study participants with lower muscle Cr concentrations tend to show the largest increases after supplementation; conversely, those with higher muscle Cr concentrations show little or no increase. Burke et al. [67] suggest that the higher starting values of the participants may have been the reason for the lack of increase in PCr and total Cr experienced by two of the three groups in this study. As is the case with d-pinitol, a very limited amount of work has examined the effects of ALA on Cr accumulation.
The herb Russian tarragon (RT) is an ethanolic extract that may have antihyperglycemic activity when combined with CM ingestion. To support this hypothesis, Jäger et al. [68] reported that RT influences plasma creatine levels during the ingestion of CM in a similar manner to glucose and PRO. The authors suggest that theoretically, ingesting RT extract prior to Cr loading may enhance insulin sensitivity and thereby promote greater Cr absorption/retention. In a follow-up study by this group, Oliver et al. [69] used a double-blind, randomized, and crossover manner to examine the effect of consuming RT during 5 days of CrM loading. Recreationally trained males ingested 500 mg of aqueous RT extract or 500 mg placebo 30 min prior to ingesting 5 g of CrM twice per day for 5 days and then repeated after a 6-week washout period. Muscle and whole-body Cr retention was assessed. Results showed that in these healthy adults, short-term CrM supplementation (10 g · day−1 for 5 days) significantly increased whole-body Cr retention and muscle free Cr content. However, ingesting 500 mg of RT 30 min prior to CrM supplementation did not affect whole-body Cr retention, muscle free Cr content, or anaerobic sprint capacity in comparison to ingesting CrM with a placebo. After showing initial promise nearly 15 years ago, the evidence on insulin mimickers and their ability to enhance muscle Cr accumulation during CrM supplementation is still scarce. At this point, more investigations are required before evidence-based recommendations, if any, can be made.
9.2.6 Creatine Salts
Cr has been combined with different organic acids to form Cr salts with the intention of using acids that will create a synergistic effect or simply improve the properties of Cr. For example, creatine salts such as citrate, maleate, pyruvate, ketoisocaproate, and orotate have been available to consumers since as the late 1990s [18]. A limitation of combining Cr with organic acids to form a Cr salt is that effective daily doses of Cr and the acid will have to match CrM to achieve meaningful physiological effects. For example, the amount of Cr in different forms of Cr salts will vary. For example, CrM contains 87.9 % creatine, whereas Cr citrate, maleate, pyruvate, ketoisocaproate, and orotate contain 66, 66, 60, 50 and 45.8 % creatine, respectively [18].
Several investigations have used Cr salts to demonstrate a performance-enhancing effect. For example, Jager et al. [70] examined the effect of the administration of two different Cr salts compared to CrM don plasma Cr concentrations and pharmacokinetics. In a balanced crossover designed study, six healthy subjects were assigned to ingest a single dose of isomolar amounts of creatine (4.4 g) in the form of CM, tricreatine citrate (TCC), or creatine pyruvate (CPY), followed by the measurement of the plasma creatine levels. Mean peak concentrations and area under the curve (AUC) were significantly higher with CPY (17 and 14 %, respectively) in comparison to CM. Although a small number of blood samples were taken, the findings suggested that different forms of creatine may result in slightly different kinetics of plasma creatine absorption. However, muscle biopsies were not taken to determine Cr accumulation, and the authors concluded that these small differences in kinetics are unlikely to have any clinically relevant effects on muscle Cr elevation during periods of Cr loading. In the second study, Jager and colleagues [71] evaluated the effect of oral CPY supplementation on exercise performance in healthy young athletes in comparison to placebo and TCC. Results indicated that 4 weeks of supplementation with these Cr salts significantly improved performance during intermittent handgrip exercise of maximal intensity and that CPY might benefit endurance, due to enhanced aerobic metabolism. Combined, these studies are the first indication that the Cr salt CPY may have comparable effects to CM. However, very few of these investigations have been able to show clear advantages over CrM. For a more detailed review of this topic, peruse Jäger et al. [18].
Despite marketing claims, novel forms of oral Cr such as Cr phosphate [42, 72], Cr serum [26], Kre-Alkalyn ® [73], Cr PEG [74, 75], and Cr ethyl ester [76] have failed to show any clear benefit or advantage over CrM. For example, creatine ethyl ester (CEE) has been purported to be a superior form of creatine in comparison to CM. To test this hypothesis [76], compared the effects of supplementing the diet with a placebo, CM, or CEE during 42 days of resistance training. Serum Cr, creatinine, and muscle total Cr content were assessed prior to and following 6, 27, and 48 days of supplementation and training. The researchers found that CEE supplementation promoted a very modest increase in muscle total Cr content, compared to the Cr accumulation shown in the CM group at days 6 and 27. In fact, study participants ingested twice the recommended dose of CEE, yet the CrM group resulted in significantly higher levels of serum Cr than the CEE group. Previous studies have shown that CEE degrades rather quickly to creatinine when exposed to low pH levels as would be found in the stomach [77, 78]. In the Spillane trial, serum creatinine levels were significantly increased in the CEE group after 6, 27, and 48 days of supplementation indicating less efficient uptake. These findings directly contradict claims that CEE is more effective in increasing muscle Cr stores.
PEG is a compound consisting of repeated chain ethylene oxide units. The water-soluble properties of PEGylation may improve absorption, half-life, and resistance to pH degradation of Cr. PEG Cr has been analyzed in two human trials, one utilizing 1.25 and 2.5 g doses of PEG compared to 5 g doses of CrM [74, 75]. Results of these studies showed that while PEG Cr might be effective, it does not provide a clear advantage or benefit compared to CrM. According to product claims Kre-Alkalyn ® is “up to ten times more powerful than ordinary Creatine.” However, the only study to examine this claim by Jagim et al. [73] demonstrated that neither manufacturers recommended doses of this buffered form of Cr (Kre-Alkalyn ® ) (1.5 g · day−1) or loading (20 g · day−1 for 7 days) and maintenance doses (5 g · day−1 for 21 days) promoted greater changes in muscle Cr content, body composition, strength, or anaerobic capacity than traditional supplementation with CrM (20 g · day−1 for 7 days, followed by a maintenance dose 5 g · day−1 for 21 days). Additionally there was no evidence that supplementing the diet with a buffered form of Cr resulted in fewer side effects than CrM. Collectively the current body of literature involving novel forms of Cr shows clearly that none are more effective than CrM at increasing muscle Cr concentrations. Other popular compounds such as HMβ and β-alanine assessed in combination with CrM did not assess muscle Cr concentrations in response to supplementation, and therefore their results are discussed elsewhere in this chapter.
To summarize this section, supplement combinations that have been shown to increase muscle Cr concentrations are presented in Table 9.1. CrM’s ergogenic potential and capacity to enhance the bioenergetics of the phosphagen system are thought to reside in the extent of Cr accumulation within muscle. This has led to increased interest in combining CrM with compounds to improve the uptake and accumulation of Cr within muscle. However, when viewed in comparison to the large body of literature that demonstrates CrM’s widespread use, safety, and performance-enhancing effects, a relatively undersized amount of work documents effective strategies and supplement combinations that may improve muscle Cr accumulation in response to supplementation. Probably due to an insulin-stimulating effect on the cellular Cr transporter, combining each dose of CrM with an insulin-stimulating CHO and PRO (~50 g of each or a total of 1 g · kg−1) appears to be a most effective strategy to improve Cr accumulation. The combination of PRO and CHO is particularly effective when smaller doses of these macronutrients are desired. The current crop of insulin-mimicking compounds such as d-pinitol ALA and Russian tarragon still has not demonstrated clear data on Cr accumulation in muscle to warrant research-based recommendations. While some of the findings on Cr salts (pyruvate in particular) are interesting, it is difficult to find evidence or a rational that warrants their use over CrM. Despite marketing claims, none of the novel forms of Cr on the market (Cr serum, Cr PEG, Cr ethyl ester, and buffered Cr) can demonstrate any clear benefit or advantage over CrM, and definitely none are able to demonstrate they promote better Cr accumulation within muscle.
Table 9.1
Supplement combinations and their effects on muscle creatine accumulation
Reference | Comparison | Assessment | Protocol | Main findings |
---|---|---|---|---|
Green et al. [59] | CrM + CHO compared to CrM only | Muscle [PCr and Cr] before and after supplementation, no exercise | 5 g CrM or 5 g CrM+ 93 g CHO (glucose) 4 × · day−1, 5 days | After 5 days, 60 % greater [PCr] from CrM + CHO (P < 0.01) |
Robinson et al. [60] | High-CHO diet with CrM vs. high-CHO diet without CrM | Muscle [PCr and Cr], one-legged cycle exercise to exhaustion preceded supplementation | 20 g CrM · day−1 5 days | After 5 days, 23 % greater increase in muscle [total Cr] in exercised limb (P < 0.01) |
Greenwood et al. [61] | CrM + CHO compared to CrM only and effervescent Cr | Whole-body Cr retention via 24-h urine samples for 4 days, 3 days of supplementation, no exercise | 5 g CrM + 18 g CHO (glucose) 4 × · day−1, or equivalent dose of CrM, 3 days | After 4 days, greater Cr retention from CrM + CHO compared to other groups (P < 0.05) (~% CrM retained 0, 60, 80, & 60 for P, CrM, CrM + CHO, & effervescent Cr, respectively) |
Preen et al. [62] | CrM + CHO compared to CrM only and CrM + exercise (E) | Muscle [PCr and Cr] before and after 5 days of intervention, one group performed exercise | CrM: 20 g · day−1 CrM + CHO: 20 g · day1 + glucose 1 g · kg−1, 2× · day−1 CrM + E: 20 g · day−1 + 60 min repeated sprints daily | After 5 days, 9 % greater increase in [total Cr] from CrM + CHO (P < 0.05) (25 % vs. 16 % & 18 % for CrM + CHO vs. CrM & CrM + E, respectively) |
Derave et al. [79] | CrM + PRO compared to CrM only and placebo (P) | Muscle [PCr and Cr] prior to and after 2-week right-leg immobilization followed by 6 weeks of right-leg resistance training | CrM: 15 g · day−1 during immobilization followed by 2.5 g · day−1 during rehabilitation CrM + PRO: CrM dose + 40 g protein and 6 g AA during training | After training, ~30 % increase from baseline in [total Cr] (right leg) in both CrM and CrM + PRO vs. P (P < 0.05) |
Steenge et al. [43] | CrM + CHO (low & high dose) compared to CrM + CHO + PRO | Insulin and whole-body Cr retention values (24 h) before and after each supplement trial (all participants completed 4 trials) | CrM (4 × 5 g) +5 g CHO +50 g CHO, +93 g CHO or + PRO + CHO (50 g each) | After 24 h PRO + CHO provided similar insulin responses and [total Cr] accumulation values (~25 %) as high-dose CHO (P < 0.05) |
Cribb et al. [63] | Compared CrM + CHO and CrM + PRO to CHO and PRO alone | muscle [PCr and Cr] before and after 11 weeks of resistance exercise | All groups: 1.5 g of supplement · kg · day−1 for 11 weeks CrM groups: 0.3 g · kg · day−1 5 days followed by 0.01 g · kg · day−1 for 10 weeks | After 11 weeks, ~10 % increase from baseline, [total Cr] after 11 weeks in both CrM + CHO and CrM + PRO groups (P < 0.05) |
Cribb et al. [80] | Compared PRO + CHO to CrM + PRO + CHO and PRO alone | Muscle [PCr and Cr] before and after 10 weeks of resistance exercise | All groups: 1.5 g of supplement · kg · day−1 for 11 weeks. CrM groups: 0.3 g · kg · day−1 5 days followed by 0.01 g · kg · day−1 for 10 weeks | After 11 weeks, ~10 % increase from baseline, [total Cr] after 11 weeks in both CrM + CHO and CrM + PRO groups (P < 0.05) |
Cribb and Hayes [67] | Compared supplement timing, CrM + PRO + CHO before and after resistance exercise, to the same supplement at times not close to training | Muscle [PCr and Cr] assessed before and after 10 weeks of resistance exercise | Dose: 1.g supplement · kg−1 2× · day−1 (CrM: 0.01 g · kg · −1) Taken immediately before and after workouts. Or twice a day 5 h outside workouts, 10 weeks | After 10 weeks, 14 % greater increase in [PCr] and 18 % greater increase in [total Cr] from supplement timing (PCr 16 % vs. 2 %; total Cr 25 % vs. 7 %, respectively) (P < 0.05) |
Greenwood et al. [69] | Compared CrM+ d-pinitol high dose (HP) and low dose (LP) as well as pre-dosing (PreP) to CrM only and placebo (P) | Whole-body Cr retention via 24-h urine samples for 4 days, 3 days of supplementation, no exercise | CrM 4 × 5 g +2 × 0.5 g d-pinitol (LP) +4 × 0.5 g d-pinitol (HP) d-Pinitol 2 × 0.5 g d-pinitol 5 days prior to and during CrM (PreP) | After 4 days, whole-body Cr retention was greater in LP and PreP compared to HP, CrM only and P (P < 0.05) (% CrM retained 0 ± 0, 61 ± 15, 83 ± 5, 61 ± 22, and 78 ± 9 % for P, CM, LP, HP, and pre-P groups) |
Burke et al. [73] | Compared ALA + CrM + CHO to CrM + CHO and CrM only | Muscle [PCr and Cr] assessed before and after 5 days of intervention | CrM: 20 g · day−1 +100 g · day−1 sucrose (CrM + CHO) +1000 mg · day−1 ALA (ALA + CrM + CHO) | After 5 days, greater increase in [PCr] and [total Cr] from ALA + CrM + CHO (P < 0.05) compared to CrM + CHO and CrM only (% increase for [PCr] 21, 0, & 0 %; [total Cr] 13.8, 2, & 4 % for ALA + CrM + CHO, CrM + CHO, and CrM, respectively) (P < 0.05) |
Spillane et al. [57] | Compared maltodextrin placebo (PLA), CrM, and creatine ethyl ester (CEE) | Serum and muscle Cr assessed days 0, 6, 27, and 48 during a 4-day per week resistance-training program in young adult males | 0.30 g/kg LBM for 5 days followed by ingestion at 0.075 g/kg LBM for 42 days. Note CEE dose was double the manufacturer’s recommendation | Higher serum Cr concentrations in CrM (p = 0.005) compared to CEE Total muscle Cr content higher in CrM (p = 0.026) and CEE (p = 0.041) compared to PLA, CrM increased total muscle Cr levels at days 6 and 27. CEE only on day 27 Serum creatinine was greater in CEE compared to the PLA (p = 0.001) and CRT (p = 0.001) and increased at days 6, 27, and 48 |
Kerksick et al. [39] | Compared creatine + pinitol (CRP) or creatine monohydrate (CR) | Whole-body Cr retention via urine samples before during and after 4 weeks of resistance training | Loading phase (i.e., 20 g/day 1 for 5 days) before ingesting 5 g/day 1 the remaining 23 days | Creatine retention increased (p < 0.001) in both groups as a result of supplementation but was not different between groups (p > 0.05) |
Jagim et al. [40] | Compared CrM with KA (Kre-Alkalyn) low dose (KA-L) and higher dose (KA-H) | Muscle Cr content 0, 7, and 28 days during the participants’ own resistance-training program | CrM 4 × 5 g/day for 7 days, maintenance (5 g/day for 21 days) KA manufacturer’s recommended dose KA-L = 1.5 g/day for 28 days. KA-H = equivalent loading to CrM | No overall differences were detected except KA-L did not increase muscle free creatine content to the same degree as loading and maintenance doses of CrM (KA-L −1.1 ± 4.3, CrM 11.2 ± 4.3 mmol/kg DW, p = 0.053 KA-L 2.4 ± 8.5, CrM 24.6 ± 8.5 %, p = 0.078 |
Oliver et al. [81] | Compared RT extract or 500 mg placebo during 5 days of CrM loading | Muscle Cr content and whole-body daily Cr retention | Double-blind, randomized, and crossover manner, 500 mg of aqueous RT extract, or 500 mg placebo 30 min prior to ingesting 5 g of CrM twice per day for 5 days, then repeated after a 6-week washout period | Whole-body daily Cr retention increased in both groups. However, the addition of RT did not enhance whole-body Cr retention or muscle free Cr content |
9.3 Supplement Combinations to Promote Muscle Glycogen
Along with the phosphagen system, glycolysis and glycogenolysis are considered to be important energy contributors during prolonged and high-intensity exercise. For example, while the depletion of CHO is considered one of the primary causes of fatigue and poor performance during prolonged, strenuous exercise [82], muscle glycogen also fuels the regeneration of ATP during short-term, high-intensity (anaerobic) exercise. During a set of 12 maximum-effort repetitions, just over 82 % of ATP demands are estimated to be met by glycogenolysis [83]. A single bout of high-intensity resistance exercise characteristically results in a significant reduction in muscle glycogen of 30–40 % [84–86]. Conversely, the synthesis and restoration of muscle glycogen is affected not only by the extent of depletion but also by the type, duration, and intensity of the preceding exercise [15–17, 82, 87, 88]. The rapid restoration of muscle glycogen stores is a critical issue for all athletes who undertake training or competition sessions on the same or successive days. In general, the faster muscle glycogen stores can be replenished after exercise, the faster the recovery process and the greater the return of performance capacity [88].
However, when considering nutritional strategies that promote glycogen synthesis and restoration, it is important to remember that high glycogen values (excess of 200 mmol · kg wet muscle weight) can be achieved without a depletion phase [87] and with as little as 24–36 h of rest and high CHO intake (10 · kg · day) [89, 90]. Additionally, even the short-term high-CHO loading (~10–12 g · kg · day) protocols utilized may not suit all athletes’ circumstances. In fact, promoting supercompensatory glycogen levels might only be of benefit to select endurance events such as time trials or where critically low (25 mmol · kg wet weight) muscle glycogen occurs [91, 92]. It is exciting to see the recent literature shifts from sports nutrition to a more pragmatic focus beyond traditional glycogen-loading diets, the generic grams per day and the often irrelevant macronutrient percentage recommendations, and addresses more contemporary issues such as rapid refueling techniques [93], fueling during restricted carb intake [94], and other strategies that may increase CHO availability [95–98].
9.3.1 Before Exercise
More recent guidelines recommend that athletes undertake their activity and daily training sessions with high-CHO availability [95, 99]. This is based on clear evidence that strategies such as elevating pre-exercise muscle glycogen stores, consuming a pre-exercise CHO meal, or eating CHO during exercise increase endurance [93, 95] and performance [96, 99]. The concept of promoting CHO availability resides in the manipulation of the amount, type, and timing in the hours or days prior to and during the session and refueling recovery between sessions to provide athletes with practical recommendations. Choices that provide a high-CHO availability contain a comparatively high amount of energy and CHO, such as glucose (other sugars, polymers in sports drinks etc.). However, the extent of CHO availability is also often reflected in the degree of fiber and processing. In general, CHO choices toward the top of the list provide the most concentrated, easily assimilated source of fuel such as cereals and grain products. They are easily identified via packaging and often an extensive ingredient list. Conversely, CHO choices with low availability generally contain a proportionally higher fiber content (such as pulses and legumes) and/or a lower amount of energy and CHO (most vegetables). For a complete list and discussion on foods that promote CHO availability, refer to Cribb [98]. Athletes are often prescribed CHO recommendations in grams per day to meet goals in their training phases. An advantage of the high or low availability classification is the clear identification and prescription of carb choices to either promote or restrict CHO availability to suit individual needs and goals during each training phase. For example, the endurance athlete can make clear, confident selections based on their grams per day prescription to promote CHO availability in the days and hours leading up to and during sessions as well as strategic refueling after strenuous endurance-based activities to optimize glycogen synthesis rates, particularly if the next bout of exercise is less than 8 h away. Conversely, many strength athletes characteristically restrict CHO intake during intense training [100, 101]. One bout of resistance exercise can decrease muscle glycogen stores in the vicinity of 24–40 % [84–86], and extensive exercise-induced muscle damage can also affect glycogen uptake and synthesis [102]. CHO availability can affect work capacity, some anabolic and muscle-specific catabolic responses. The reader is recommended a more detailed discussion and application of CHO availability [98].
Pre-exercise meals and supplementation have been a considerable focus of exercise research because of its impact on substrate utilization [103–105]. However, the respective cases for high- or low-glycemic index pre-exercise meals to optimize performance appears to be equivocal [106, 107] and might be negligible, as the opportunity to consume CHO during exercise seems to nullify any glycemic characteristics of pre-exercise meals [108–110]. It is presumed that CHO immediately before and during exercise can provide an additional fuel source that may spare muscle glycogen, prevent low blood glucose concentrations, attenuate effort perception, and benefit the central nervous system as well as promote glycogen resynthesis during low-intensity periods [108–113]. Probably for these reasons, the investigation of CHO “fuel mixes” during exercise has received considerable attention.
9.3.2 During Exercise
Throughout the 1990s it was presumed that the oxidation (utilization) rate of exogenous CHO was a maximum of 60 g · h—larger amounts would likely not be metabolized and may cause gastrointestinal distress. However, most recent work suggests CHO intake rates can vary greatly among athletes, from 6 to 136 g · h, and variations at both ends of this scale can result in nausea and flatulence but also winning performances during ultra-endurance races [109]. Studies that have systematically tracked oxidation rates of various CHO sources and combinations now reveal that the rate limiting step in the oxidation of ingested CHO could be intestinal absorption, with limits on absorption of glucose in its various forms by the sodium-dependent glucose transporters (e.g., fructose via GLUT5 and glucose via SGLT1) [79, 114]. For example, limit on the absorption of glucose in its various forms via the sodium-dependent glucose transporter SGLT1 is ~1 g · min. When glucose is combined with fructose (a CHO with a different transporter), rates of ingested CHO can exceed 1.5 g · min. It appears that exogenous CHO oxidation to maintain power output when glycogen becomes depleted converges at a CHO ingestion rate of 80–90 g · h at least when glucose and fructose are co-ingested in a 2:1 ratio [97]. Generally, fluids/gels/bars that contain “multiple transporter CHOs” appear to be well tolerated [115, 116], promoting greater oxidation efficiency (less CHO remaining in the gastrointestinal tract) [79, 114–117] and delivering high rates of CHO [116, 118] and performance benefits when compared to glucose alone, particularly in activities lasting 3 or more hours [119, 120]. Based on this new information on CHO supplement combinations, new guidelines to promote individual experimentation with CHO intakes of up to 90 g · h in ultra-endurance sports have been proposed [93].
9.3.3 Post-exercise Refueling
Rapid recovery for subsequent performance is a prerequisite for effective training and competition, and this predominately relies upon the restoration of glycogen stores. Rapid restoration of glycogen stores is essential if exercise is required again within 24 h [95]. However, even for daily exercise, there is the real possibility that muscle glycogen would not be adequately replenished unless strategies are implemented. For example, pre-exercise muscle glycogen stores of endurance athletes are usually ~600 mm · kg · dry weight, and exhaustive exercise can deplete stores to 30–55 mm · kg dry weight [87, 121, 122]. Post-exercise glycogen synthesis rates can vary from ~5 to 58 mm · kg · dry weight · h [122–124] depending on the type and amount of fuel consumed. Therefore, maximum glycogen restoration could be achieved efficiently within 5–6 h or could take up to 48 h, depending upon the strategies (or the lack of) implemented. The process of refueling does appear to be highly dependent on the provision of nutrients. For example, a delay in CHO intake post-exercise could result in a decrease in glycogen synthesis of up to 50 % [123]. Conversely, strategies that involve the consumption of rather high intakes (1.2 kg · h) report the highest rates for glycogen synthesis [93–95]. However, these amounts might not be practical for all athletes.
Combining a lower CHO intake with PRO supplements can assist with maximizing glycogen stores. Reported rates of muscle glycogen resynthesis across nine studies that have compared muscle glycogen storage over 2–6 h post-exercise with varied rates of CHO intake suggest that the addition of PRO to CHO intake (less than 1.2 g · kg · h) will enhance muscle glycogen synthesis [94]. CHO to PRO ratios of 4:1 have been suggested for refueling [93]. However, other ratios such as 2:1 or 3:1 may provide similar benefits, at least with regard to DOMS and markers of skeletal muscle damage [124]. In general, it appears that the addition of PRO to a CHO supplement will increase the rate of muscle glycogen storage during the hours immediately after exercise, particularly if the supplement contains a low to moderate amount of CHO. The combination of PRO and CHO may accelerate the rate of muscle glycogen storage possibly by activating glycogen synthesis by two different mechanisms. Firstly, this combination may raise plasma insulin levels beyond that typical of CHO alone, which may augment muscle glucose uptake and activate glycogen synthase via insulin-dependent and insulin-independent pathways [88]. Thus, these combinations have an additive effect on the activity of this enzyme. Glucose, sucrose, and glucose polymer supplements as well as high-CHO availability whole-food sources are an effective means of replenishing muscle glycogen. However, the type of PRO (or amino acids) that may be best to combine with CHO has received less attention. Studies that have reported a beneficial impact on muscle glycogen synthesis from the addition of PRO to a CHO supplement have utilized dairy sources [88, 125, 126] such as whey isolates [88, 127] and hydrolyzed PRO supplements in conjunction with insulin-promoting amino acids (AA) such as leucine and phenylalanine [128, 129]. In fact, studies that have examined this area directly suggests that insulin responses to PRO–CHO supplementation may be positively correlated with plasma concentrations of AA such as leucine, phenylalanine, and tyrosine [129]. Therefore, the concentration of certain AA within the supplement may underline its ability to stimulate insulin and, therefore, muscle glycogen restoration.
CrM, and more recently caffeine, has been documented as possible synergists that act with CHO to aid in glycogen synthesis rates [122]. In fact, Pedersen et al. [122] demonstrated the combination of CHO + caffeine (2 × 4 mg · kg) post-exercise can improve glycogen synthesis rates to a greater extent than CHO + PRO or CHO alone. In fact, the glycogen synthesis rates for this combination (~58 mmol · kg dry weight · h vs. 38 mmol · kg dry weight · h for CHO only) are one of the highest recorded responses for post-exercise CHO consumption [122]. Taylor et al. [130] recently utilized a similar post-exercise CHO + caffeine timing strategy and reported a significant improvement in a subsequent bout of high-intensity interval running [130]. However, the benefits need to be considered with associated side effects. Large doses of caffeine can produce nausea and disrupt sleep.
As discussed previously, supplementation with CrM promotes an ergogenic effect by enhancing PCr availability in muscle. However, another ergogenic effect from this supplement appears to be its positive impact on muscle glycogen storage. Seven studies have measured muscle glycogen levels in humans after CrM supplementation, and six have reported a stimulatory effect [53, 60, 131–134]. Robinson et al. [53] first showed that CrM supplementation in conjunction with a high-CHO diet for 5 days (after a bout of exhaustive exercise) resulted in 23 % greater increase in muscle glycogen as compared with a high-CHO diet without CrM. Nelson et al. [131] reported that loading with CrM for 5 days enhanced a subsequent 3 days muscle glycogen-loading protocol by 12 %. Op ‘t Eijnde et al. [132] demonstrated that supplementation with CrM (20 g daily) had no effect on muscle glycogen stores during 2 weeks of leg immobilization. However, further administration (15 g daily) did enhance muscle glycogen levels (by 46 % greater than placebo) during 3 weeks of subsequent strength training. In a follow-up study that involved a similar protocol (and a 6-week training phase), these researchers reported that supplementation with PRO (46 g) combined with CrM augmented post-training muscle glycogen by 35 % greater than placebo (but not CrM alone) [133, 134]. Van Loon et al. [134] demonstrated that a 5-day CrM-loading phase augmented muscle glycogen by 14 % compared with no change in the placebo group. Furthermore, this study confirmed a significant correlation between changes in muscle Cr (mean increase of 32 %) and muscle glycogen during the loading phase. This substantiates other work [131–133] that suggests significant increase in muscle Cr is a prerequisite for enhanced muscle glycogen storage [28]. Supplementation with CrM + CHO or PRO during exercise increases muscle glucose transporter (GLUT-4) expression and glycogen storage [132, 133]. Treatment with CrM has also been shown to increase total body water including intracellular cell volume [135]. Changes in cell volume (cellular water content) have been shown to influence glycogen levels [136]. Therefore, the ability of CrM to influence GLUT-4 biogenesis and/or regulate cell volume may explain its beneficial impact on muscle glycogen storage. Overall, the findings of these studies suggest that increasing muscle Cr (by ~20 %) via supplementation ensures a beneficial impact on glycogen storage. However, for some athletes, careful consideration is needed when contemplating the addition of CrM. For example, loading with CrM characteristically results in a 1–3-kg gain in body weight (lean mass) [14, 20–22, 24, 25]. This added mass may offset any potential ergogenic benefit that might be achieved via boosting muscle glycogen stores. Therefore, in sports where any gain in body weight may disadvantage the athlete, the combination of PRO–CHO (without CrM) may be a more prudent choice to promote muscle glycogen. However, for all athletes, alongside more efficient glycogen restoration, another important advantage of PRO–CHO post-exercise supplementation is this combinations’ well-documented effect on synthesis and muscle anabolism.
9.4 Supplement Combinations to Enhance Muscle Anabolism
Any favorable adaptive change in muscle must involve alterations in protein turnover. That is, the difference between rates of muscle protein synthesis (MPS) and muscle protein breakdown (MPB) determines net protein balance (NPB) [137]. A single bout of resistance exercise results in the acute stimulation of MPS 50–100 % above basal values which peaks within 3 h and remains elevated at a diminishing rate for up to 48 h post-exercise [138, 139]. NPB after resistance exercise remains negative, until nutrition, namely, a PRO source, is provided. The stimulation of MPS is the critical regulatory event in protein turnover that underlines changes in muscle mass [140]. The majority of acute-response investigations have shown that the consumption of amino acids/PRO close to resistance exercise results in the amplification of MPS, and the effect appears to be synergistic rather than simply additive [141, 142]. A CHO supplement (glucose, other sugars, and polymers) alone, before, during, or after resistance exercise, does not appear to alter the MPS [143] response, but it may reduce myofibrillar breakdown [144, 145] and circulating cortisol which can correspond with a greater increase in muscle fiber hypertrophy [146, 147]. Interestingly, the combination of a CHO supplement (such as glucose) with essential amino acids (EAA) (6–40 g), before or after resistance exercise, provides a synergistic effect on MPS and NPB that is much greater than either macronutrient alone [148–150]. In fact, when this combination is consumed 1 or 3 h after a single bout of resistance exercise, MPS rates were shown to increase up to 400 % above pre-exercise values, the highest value ever recorded [142]. While earlier investigations reported positive effects from amino acids, it is now clear that intact PRO supplements such as whey, casein, soy, and even whole milk evoke an anabolic response that can be similar in magnitude to free form amino acids [151, 152]. These acute-response investigations paved the way to more recent work that has examined the interaction of PRO–CHO supplementation on the molecular events associated with the activation of MPS.
9.4.1 Supplement Timing
For instance, the mTOR complex and its downstream mRNA translational signaling proteins (p70S6K, eIF-2B, and 4E-BP1) appear to be the focal molecular intersection point in the cascade that results in very high rates of MPS [153]. The EAAs [154] and particularly the branched-chain amino acid (BCAA) [155] stimulate MPS independently, directly via activation of this complex. Supplement timing with PRO–CHO ensures a higher activation of molecular events associated with increased MPS rates which is thought to underline improved adaptations from exercise, and these benefits are not exclusive to strength athletes [156–161]. For example, via the same molecular signaling pathway, PRO–CHO supplement timing stimulates a greater increase in MPS in other high-intensity anaerobic exercise, such as sprints [157]. This strategy also selectively stimulates a greater increase in myofibrillar MPS following prolonged cycling, and this increase appears to be mediated via the mTOR complex [153]. A higher activation of the mTOR signaling pathway, via supplement timing with PRO–CHO, not only modulates MPS but also upregulates glycogen synthesis [159] to improve recovery and subsequent exercise performance [160]. Ferguson-Stegall et al. [156, 160] reported that a PRO–CHO supplement (in the form of low-fat chocolate milk) alters the phosphorylation levels of this signaling cascade in a manner that accelerates muscle glycogen synthesis during recovery to improve performance in a subsequent bout of cycling exercise (see Sect. 8.1: Delicious Combination). Therefore, supplement timing with PRO–CHO, in close proximity to aerobic and anaerobic exercise training, results in the higher activation of the molecular signaling pathways that regulate muscle and glycogen synthesis. Additionally, strategies that promote and maintain muscle glycogen during exercise programs may provide the best chance of maximal activation of the signaling pathways that stimulate MPS during resistance exercise.
It is clear that the strategic intake of PRO–CHO before and/or after intense exercise not only augments MPS, most importantly it shifts net protein balance to a positive state. This anabolic response can be at least partly attributed to changes in the anabolic hormonal response pattern of insulin. This PRO–CHO-induced stimulation of insulin is important; it improves the anabolic response by increasing AA uptake and decreases the rate of muscle protein breakdown [142, 143, 145]. Despite over 15 years of investigations, the research on supplementation’s ability to modulate anabolic hormones, such as growth hormone, IGF-1, and testosterone in a way that may lead to changes in strength and muscle mass, is at best equivocal [162–165]. More recent work suggests transient increases in endogenous anabolic hormones do not enhance fed-state anabolic signaling or MPS following resistance exercise and quite possibly have little to do with strength and hypertrophy outcomes [166].
The EAAs and particularly the BCAAs stimulate MPS independently, via a different pathway to insulin [159]. The BCAA leucine has a controlling influence over the activation, but not the duration, of MPS [167]. Increasing the leucine concentration of a PRO serving (to ~40 %) can increase resting MPS [168] and post-exercise [169] as a high rate of MPS from feeding is the underlying mechanism for changes in muscle mass [140]. This lead to a flurry of investigations that assessed the effects of adding leucine to PRO supplements in an attempt to augment MPS rates. However, several research groups have failed to achieve a greater elevation of MPS by adding leucine to a moderate dose of high-quality PRO (10–30 g) at rest [170] and post-exercise [171, 172]. Glynn et al. [170] reported no added benefit for resting human MPS in response to an optimal dose (10 g) of EAA that provided a higher concentration of leucine (3.5 g compared to 1.8 g). In fact, a low dose of whey PRO (6 g) with added leucine or all other essential amino acids (to the equivalent of 25 g of whey PRO isolate) was not as effective as 25 g of whey PRO isolate alone at sustaining increased rates of MPS post-exercise [172]. These findings are important because they show that an EAA profile from high-quality PRO is sufficient for stimulating MPS in humans and additional leucine does not provide added benefit to MPS. Nevertheless, the ability of various PRO/AA supplement combinations to augment the anabolic response to exercise may well be population specific [173]. Combining PRO with leucine to optimize MPS and anabolism may be a good strategy for populations such as the elderly but also military where larger PRO servings (20 g or more) are difficult to incorporate into a feeding plan [174].
9.5 Combinations that Enhance Aerobic/Anaerobic Performance
Caffeine is a naturally occurring substance that is a widely consumed in a variety of forms. Caffeine produces multiple physiological effects throughout the body including increased catecholamine release and fat metabolism, resulting in glycogen sparing, increased intracellular Ca2+ release, inhibition of cAMP phosphodiesterase, and antagonism of adenosine receptors [175]. Several studies have demonstrated an improvement in exercise performance in submaximal endurance activities [81, 175–178], while its potential ergogenic effect in acute, high-intensity exercise is less clear in untrained individuals [175]. However, in a systematic review, studies using trained subjects and models specific to intermittent sports activity support the idea that caffeine is potentially ergogenic with anaerobic exercise [80]. Ephedrine was used as a CNS stimulant in China for centuries before its introduction to Western medicine in 1924 [179]. Ephedrine and its related alkaloids (mostly pseudoephedrine) are sympathomimetic agents which stimulate the sympathetic nervous system, increasing circulating catecholamines [180]. A number of studies have reported beneficial effects on exercise performance using ephedrine as the supplement [181, 182], whereas limited studies have reported benefit utilizing the related alkaloids such as pseudoephedrine [183, 184]. This is most likely due to ephedrine’s direct adrenoceptor stimulating actions [185] and, therefore, is approximately 2.5-fold more potent than pseudoephedrine [183]. Though both caffeine and ephedrine have demonstrated independent ergogenic effects on exercise performance, research published from Doug Bell and Ira Jacob’s lab at the Defense and Civil Institute of Environmental Medicine in Canada has reported in several instances that caffeine–ephedrine mixtures confer a greater ergogenic benefit than either drug alone [181, 186–189]. In a series of studies performed by Bell and Jacob [181, 186–188], enhancement of performance was observed during various exercise modalities: submaximal steady-state aerobic exercise [188], short- and long-distance running [186, 188], and maximal anaerobic cycling [187]. The caffeine–ephedrine mixture was consumed 1.5 to 2 h prior to exercise with a dosage range of 4–5 mg/kg for caffeine and 0.8–1 mg/kg for ephedrine [181, 186–188]. However, it should be noted that dosages above this were shown to illicit negative side effects such as vomiting and nausea during the exercise test. With this in mind, Bell and colleagues recommended using the lower dosage of 4 mg/kg and 0.8 mg/kg for caffeine and ephedrine, respectively. Importantly, the lower dosage still provided an ergogenic effect similar in magnitude to those reported previously using the higher doses [190].
Notwithstanding the independent effects from caffeine and ephedrine on metabolic and cardiovascular responses during exercise, no ergogenic effects on exercise performance were observed. However, when combined, exercise performance was significantly enhanced in a variety of exercise modalities. Researchers suggested that this is most likely due to ephedrine’s effect on arousal (i.e., decreasing rating of perceived exertion during exercise), combined with caffeine’s ability to enhance muscle metabolism [188, 189, 191]. Indeed Williams et al. [192] were also able to show increased alertness and enhancement of mood following ingestion of similar doses of caffeine (300 mg) and ephedra (60 mg) 45 min prior to exercise testing. However, despite these improvements, caffeine and/or ephedra was unable to enhance muscle strength, muscle endurance, and peak anaerobic power. Given the beneficial effects of caffeine and ephedrine have predominately been observed in studies involving the Canadian military, it is clear that further research is needed to examine the practical application in recreational athletes and untrained individuals. However since 2006, all dietary supplements containing ephedrine alkaloids are illegal for sale in the United States [193]. Therefore, until ephedrine and ephedrine alkaloids are made legal again, very few studies if any are investigating the performance-enhancing effects of caffeine–ephedrine mixtures.
Recently, other ergogenic aids such as sodium bicarbonate (NaHCO3) and carbohydrate have also been used in combination with caffeine [194–196]. A recent systematic literature review examined the benefit of co-ingestion of caffeine and carbohydrate ingestion on endurance-exercise performance [197]. Criteria for inclusion were studies that used human subjects performing an endurance-exercise performance task and included both a carbohydrate and carbohydrate–caffeine condition. Based on effect size analysis, the authors concluded that co-ingestion of carbohydrate and caffeine provides a significant but small effect to improve endurance performance compared to carbohydrate alone. This was also confirmed in a recent study that showed that ingesting carbohydrate and caffeine 1 h before, prior to and during an intermittent sprint test (modified version of the Loughborough Intermittent Shuttle Test), was effective at transiently reducing fatigue and rating of perceived exertion while maintaining higher glucose levels at the final stages of the exercise test [198]. Conversely, co-ingestion of caffeine and carbohydrate was unable to improve high-intensity sprint cycling performance or reduce fatigue in active males. Interestingly, the combined caffeine and carbohydrate group demonstrated higher cortisol levels during the prolonged high-intensity intermittent exercise suggesting higher catabolism [196]. In contrast to the potential benefits of combining caffeine with carbohydrate, the combination of caffeine with sodium bicarbonate seems to provide no added benefit [194, 195]. Two recent studies used trained individuals to examine the combined effects of caffeine and sodium bicarbonate on high-intensity cycling performance and 2,000-m rowing performance [194, 195]. Despite independent benefits of supplementation, co-ingestion provided no greater advantage. Interestingly, while both studies used the same dosage of sodium bicarbonate (0.3 g/kg body mass), only one study demonstrated negative side effects [194].
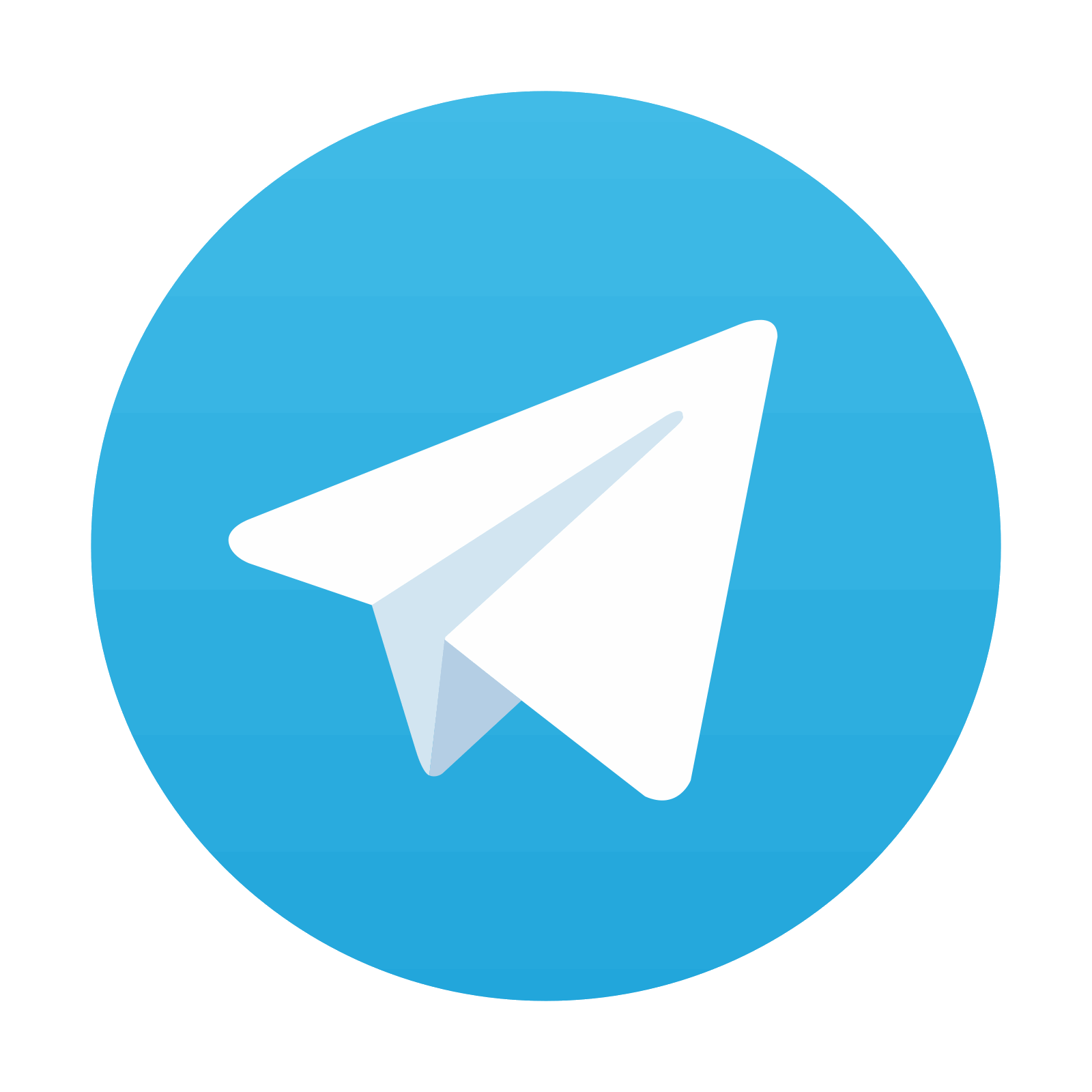
Stay updated, free articles. Join our Telegram channel
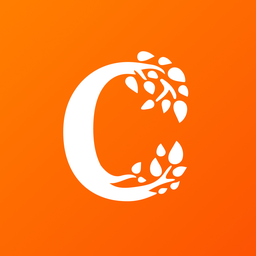
Full access? Get Clinical Tree
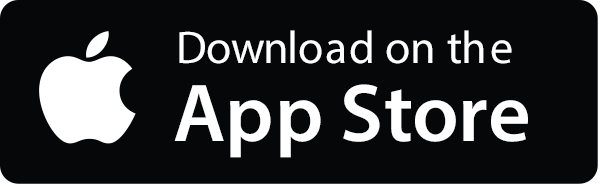
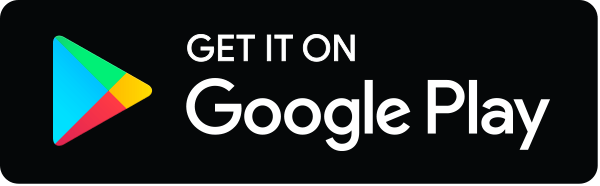