Fig. 7.1
Left: Setup to initiate ACL rupture injuries, consisting of custom platens designed to hold the knee and ankle joints, a load cell, and a tube for administering anesthesia, assembled on an electromagnetic materials testing instrument (Bose ElectroForce 3200). Top right: Close-up of the top platen showing a ruler calibrated in inches. Bottom right: Close-up of the bottom platen showing a shallow cup to hold the knee, with a groove on one side to accommodate the thigh
Mice are anesthetized using isoflurane and then placed onto the loading device with continued administration of isoflurane anesthesia, as shown in Fig. 7.1a. The right ankle and knee are placed into the upper and lower platens, and a pre-load of 0.5–1.5 N is applied to hold the leg in place. To create the ACL rupture injury, the upper platen is lowered to a target axial compressive load of 12 N, or a target displacement of 1.7 mm, depending on the type of ACL disruption desired (discussed in detail below). The axial loading causes a transient anterior subluxation of the tibia relative to the distal femur, in effect moving the tibia in the direction that the anterior cruciate ligament stabilizes. A release of compressive force indicates failure of the ACL, which reproducibly occurs at 8–11 N compressive load for most mice (Fig. 7.2). After the target of 12 N or 1.7 mm is reached, the platen is returned to its initial position and the knee joint is restored to its native orientation. The entire procedure takes less than 5 min including anesthetization, with tibial compression loading requiring only about 6 s, with injury typically occurring after about 2 s (Fig. 7.2). Mice are removed from the setup gently and given a dose of analgesic while still anesthetized. To date, all animals have survived the injury with no fractures to the long bones, and they are typically mobile soon after recovering from anesthesia and show only very mild, if any, changes in apatite, behavior, or activity.
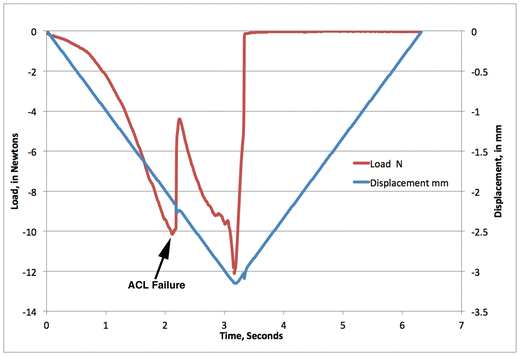
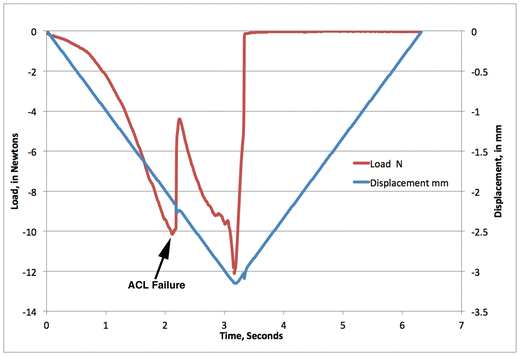
Fig. 7.2
Typical loading profile to initiate ACL rupture by avulsion fracture. The blue line indicates displacement of the top platen that holds the ankle, and shows a slow constant movement from 0 mm down to −3 mm, and back to 0 mm. The red line is the readout from the load cell under the knee. It shows that as the ankle is pushed downwards, there is a steady increase in load until just over 10 N, at which point the rapid release of compressive force (shown by the arrow) indicates disruption of the ACL
Immediate Assessment of Injury
Experienced orthopedic surgeons that were blinded to the injury status assessed the immediate effect of injury semiquantitatively. All injured knees were correctly identified as injured, but one (of 5) uninjured was also identified as injured. The most commonly observed indicators of joint injury were increased anterior/posterior translation and external rotation of the knee, with some minor swelling and hemarthrosis [19]. Based on the extent and the type of laxity observed, the damage to the joint was considered consistent with ACL rupture, but not indicative of damage to either the medial or collateral ligaments. Analysis by either standard or contrast-enhanced microCT imaging confirmed that ACL rupture was present in all injured knees that were imaged [19, 20]. Damage to other joint structures such as the PCL, meniscus, patella, collateral ligaments, was not obvious by high-resolution contrast-enhanced microCT [20].
The mode of ACL rupture depends on the rate of axial compression [20]. A relatively slow compression rate of 1 mm/s causes ACL disruption with an avulsion fracture, in which the ACL is disrupted at the site of insertion into bone, and pulls a segment of bone usually from the posterior femur into the joint cavity. Clinically, ACL injuries with an avulsion fracture are more common in children than in adults. A much faster loading rate of 500 mm/s causes a midsubstance disruption of the ligament with no evidence by microCT of an avulsion fracture. Clinically, midsubstance ACL tears are more common in adults. On a technical note, due to limitations in the software and data acquisition rates of the materials testing setup used in these studies, we used a target load of 12 N as a trigger to stop the injury only with the slow injury rate of 1 mm/s. In the fast injury rate (500 mm/s) it became necessary to use a displacement of 1.7 mm as a trigger to stop compression because of overshoot of the target force. In both cases, the loads required to induce injury were very similar, ranging from 8 N to 11 N in the majority of animals. Another technical limitation of our software and hardware setup is that the downward motion of the upper platen is not programmed to stop automatically when the ACL rupture injury occurs. Although we would ideally like to experimentally control this aspect of the ACL rupture injury in our mouse model, it is not necessary to do so in order to mimic clinically relevant human ACL injuries. In our system, the downward motion continues until a trigger point of either 12 N or 1.7 mm is reached. In general, for most of the assays with which we measured injury response, the fast injury midsubstance ACL tears induced very similar but somewhat milder injury responses than the slow injury avulsion fractures.
Serum Markers of OA Progression
Biomarkers of OA progression and bone remodeling can be measured from synovial fluid, blood, or urine, and are used as confirmatory measures of PTOA progression. Cartilage Oligomeric Matrix Protein is one of the more promising OA biomarkers, and serves as a marker of the earlier stages of OA in which cartilage is still present and cartilage turnover is elevated. We found that ACL rupture caused statistically significant increases in the serum levels of COMP within 1 day after injury, and COMP levels remained elevated at all time points until at least 8 weeks after injury (except the 4-week time point, which trended higher but not significantly) [19]. A serum marker of bone resorption (CTX-I) was significantly increased at 7 and 14, but not 56 days after ACL Rupture injury. At the same time points, a marker of bone formation (P1NP) remained unchanged [21]. Together these results suggest that in the earlier stages after joint injury, ACL rupture-induced changes in bone turnover are primarily caused by changes in bone resorption rather than formation, and that elevated cartilage turnover occurs throughout the first 8 weeks after injury.
Histological Assessment of OA Progression
Histological assessment of joints injured by ACL rupture revealed deterioration of cartilage and osteoarthritic changes consistent with PTOA progression [19]. At the early time points, grading by a veterinary pathologist revealed that injury caused synovial hyperplasia, inflammation, and fibrosis. Cartilage damage was only mild at early time points (Fig. 7.3), but by 8 weeks significant loss of proteoglycan was observed. There was fissuring of the articular cartilage, frequent loss of the surface lamina and the flattened chondrocytes of the superficial zone, and atrophy of articular chondrocytes. Blinded histological grading of the 8-week sections revealed that injury caused significant increases in the OARSI score [22] at the medial tibia, medial femur, and lateral femur. No differences in OARSI scores were measured at the femoro-patellar joint or the underlying surface of the femur.
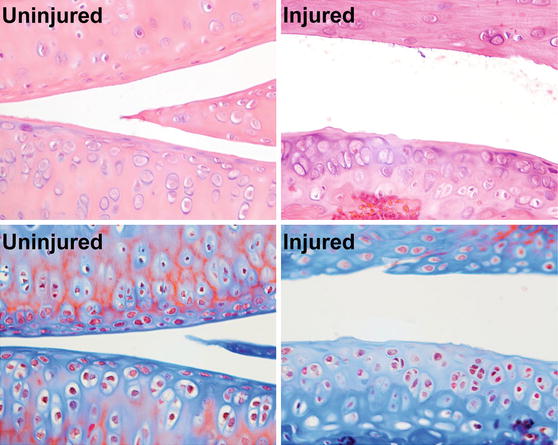
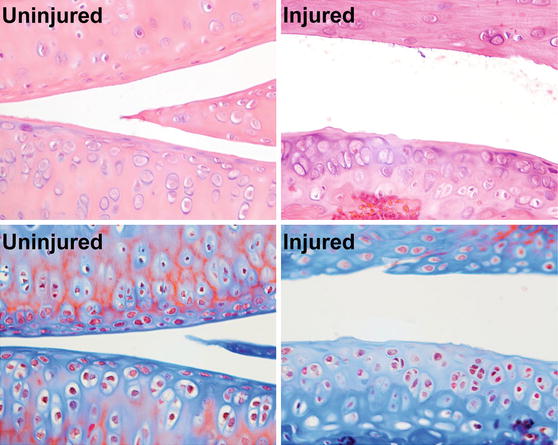
Fig. 7.3
Histological assessment of cartilage damage at 8 weeks after injury, showing injury-induced loss of surface lamina and flattened superficial chondrocytes, fibrillations in the cartilage, and cluster formation in the middle or deep layers
Radiographic Assessment of Injury Response
ACL rupture through avulsion fracture caused a rapid and substantial loss of subchondral trabecular bone volume very early after injury as measured by microCT. This trabecular bone loss became significant within 3 days after injury, and reached a maximum of approximately 40 % loss after 7 days compared to the contralateral uninjured knee. This initial remodeling phase was followed by a partial recovery of bone volume. At the 4- and 8-week time points after injury, a new but lower steady-state bone volume was reached that was approximately 80 % of the day 1 volume [19]. This lower bone volume was maintained out to 12 and 16 weeks [20]. The loss of subchondral bone volume was somewhat less severe in the midsection ACL rupture injury than in the ACL avulsion fracture (−20 % loss versus −31 % at day 10). However, this difference in bone turnover between the modes of ACL disruption was only seen at the early time points. The lower steady state bone volume reached at later time points was not statistically different between injuries created by midsection ACL rupture or ACL avulsion fracture.
We were somewhat surprised by the rate and extent of the early bone remodeling after the ACL rupture injury. Locally, a 40 % loss of subchondral trabecular bone after 7 days is a significant biological event, which alone may have consequences for the health of the joint over time. Bisphosphonates such as alendronate are specific inhibitors of osteoclast-mediated bone resorption. Given the substantial bone remodeling induced by the ACL rupture injuries, we tested whether treatment with alendronate would attenuate this response, with a secondary hypothesis that this may protect against cartilage degradation and osteoarthritis progression. We found that high doses of alendronate did prevent the short-term loss of subchondral trabecular bone volume, but did not inhibit the osteophyte formation at the later time points, or the cartilage degeneration induced by the ACL rupture injury [21].
Osteophyte formation is a hallmark of clinical OA. Osteophytes are newly formed fibrocartilage and bone growths that are prevalent at the peripheral margins of joints, and at the interface between cartilage and periosteum [23]. The ACL rupture injury models described here cause substantial nonnative new bone formation that is readily detectable within 10 days after injury, perhaps even earlier [20]. Much of this injury-induced new bone formation appears to be osteophyte formation, and thus the model reproduces aspects of clinical PTOA. In addition, a portion of the new bone formation may be enthesophytes, or new bone forming at the insertion sites of ligaments to the bone, specifically around the collateral ligaments (personal communication, Chris Little). To date we have not rigorously characterized the nonnative bone to differentiate whether it is primarily osteophytes or enthesophytes, but we suspect that both occur in response to the ACL rupture injuries. Interestingly, the milder midsection ACL tear injury tends to produce slightly greater nonnative bone volume at the later time points than ACL avulsion fractures, which is in contrast to the generally milder response to the midsection tear. While the joint injuries caused an initial destabilization of the joint (as quantified [20] by anterior–posterior joint laxity), osteophyte formation appeared to correlate with a re-stabilization of the joint, albeit with a much reduced range of motion [20].
Sclerosis of the subchondral bone plate is also a hallmark of clinical OA. The sclerosis involves remodeling and hardening of the subchondral bone plate in early OA, often accompanied by an advancing tidemark of calcified cartilage, and decreased subchondral vascularity [23]. Analysis of the subchondral bone at the proximal tibia in our model of ACL rupture revealed that the injury induced a significant thickening of the subchondral bone plate [20], where we observed increases of 20–26 % in cortical thickness in both ACL midsection tear and avulsion fracture injuries at the 12- and 16-week time points. This is in contrast to the partial medial meniscectomy (PMM) surgical injury model, in which osteophyte formation and subchondral sclerosis was not seen by microCT scans until 20 weeks after surgery [24]. In our studies, all injured knees showed a similar extent of subchondral bone sclerosis, independent of whether the mode of ACL rupture was midsection tear or avulsion fracture.
In summary, the nonsurgical ACL rupture model includes many aspects of clinically relevant post-traumatic osteoarthritis. The immediate mechanical damage resulting from the ACL rupture injury is primarily limited to the ACL itself, which can occur through either midsection tear or avulsion fraction depending on the loading rate. The injury destabilizes the joint, and initiates a short-term biological response that includes mild inflammation, mild synovial hyperplasia, fibrosis, and a rapid remodeling of the subchondral trabecular bone. Longer-term outcomes include hallmarks of OA progression such as cartilage fibrillation, loss of superficial zone chondrocytes and cartilage proteoglycan content, subchondral bone sclerosis, and osteophyte formation. At the later time points joint stability is somewhat restored, perhaps because of the extensive osteophyte and ectopic bone formation, but the restored stability is at the expense of range of motion.
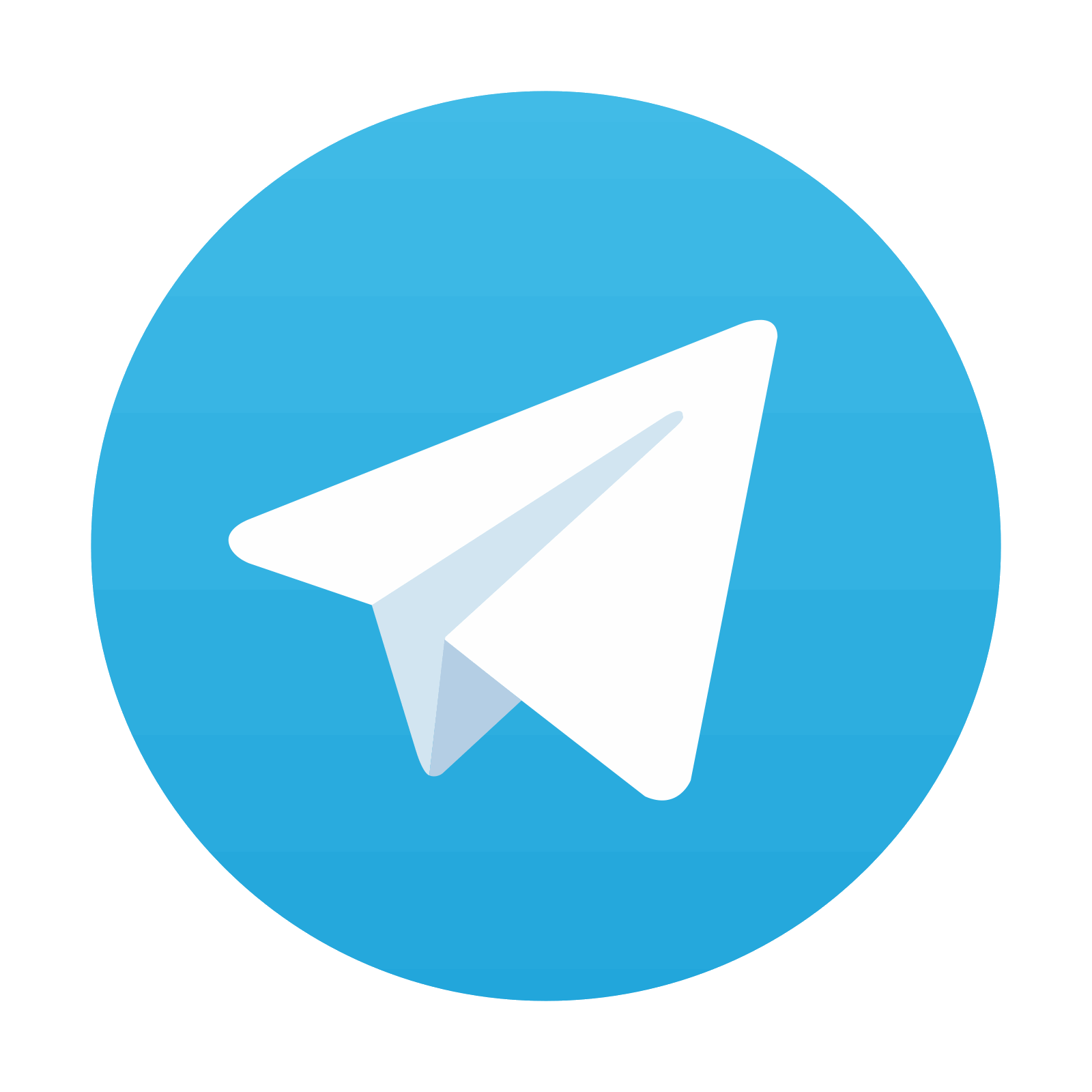
Stay updated, free articles. Join our Telegram channel
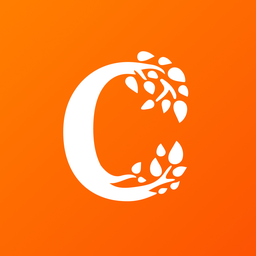
Full access? Get Clinical Tree
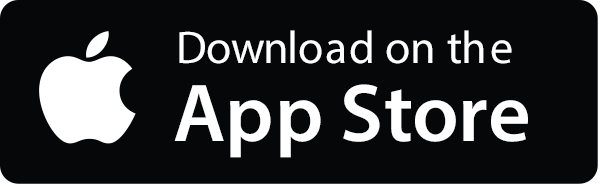
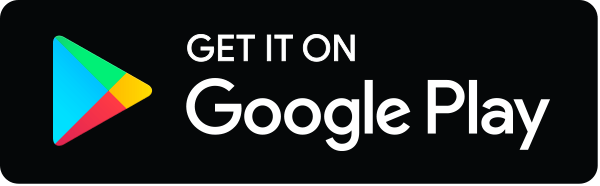