Injury description
AIS
Pulmonary contusion
3
Fracture <3 ribs
1–2
Flail chest, unilateral
3
Blunt cardiac injury, minor ECG changes
3
Torn descending thoracic aorta
4–5
Epidemiology
Thoracic injury is the primary cause of death in about 1 of 4 patients who succumb to trauma and contributes to the death of another 1 out of those 4 [2]. Most mortality directly attributable to chest trauma occurs very early (within minutes of injury) due to major cardiovascular disruption or major lacerations of the tracheobronchial tree. Excluding these early deaths, less than 5 % of patients with blunt thoracic injuries will require an operative intervention in the chest. For example, in a study of over 1,500 patients with combined blunt thoracoabdominal injuries, only 4.3 % of patients underwent thoracotomy (excluding resuscitative thoracotomies) [3]. For practical purposes, tube thoracostomy is the most invasive thoracic procedure needed in the vast majority of patients. Despite the rarity of operative intervention, patients with major chest injuries frequently have major cardiopulmonary dysfunction.
Blunt mechanisms of thoracic injury predominate in most centers, with motor vehicle accidents accounting for the vast majority. Penetrating injuries are less common and are likely to be of limited interest to those clinicians enjoying this book; they will not be discussed further. Blunt chest injuries usually occur in association with multiple injuries to other anatomic regions. Indeed—patients with major thoracic injuries typify the multiply injured patient. For example, in a 1987 study of over 500 patients admitted to the Maryland Institute of Emergency Medical Services with blunt chest injuries, only 16 % of patients had injuries limited to the chest [4]. In the Quebec trauma registry, approximately 25 % of patients with chest trauma had concomitant abdominal injuries [5]. The structures most commonly injured in the chest are the ribs, the pleura, and the lung. Major cardiac and vascular injuries, while certainly important, are in fact uncommon in patients who survive the initial insult. Blunt esophageal injuries are vanishingly rare. A summary of the distribution of injuries from three large studies is provided in Table 14.2.
Table 14.2
Relative frequency of injuries in the chest in three large published series
Injury | Cited frequency (%) |
---|---|
Rib fractures | 35–64 |
Pulmonary contusion | 16–30 |
Hemo-/pneumothorax | 11–50 |
Flail chest | 5–10 |
Heart/great vessels | 2–6 |
Given that thoracic injuries are rarely an immediate threat to life in the patient who survives the initial insult, the primary challenge for the clinician is optimizing supportive care of the cardiopulmonary system and preventing pulmonary complications such as pneumonia, ARDS, fat emboli syndrome, and prolonged ventilator dependence. The sequelae of both direct and indirect cardiopulmonary injury can substantially complicate the care of multiply injured patients. In the Hannover experience, outcome was described in 278 multiply injured patients with chest trauma (ISS > 15 and Chest AIS >2, excluding severe brain injuries) [6]. They found that length of stay averaged 33 days and rates of pneumonia and ARDS and multiple organ failure (MOF) were 22 and 13 %, respectively. In general, then, given the likelihood of prolonged and complex hospital course, the optimal care of a patient with orthopedic injuries and concomitant thoracic injuries involves proper risk assessment (is it safe to take this patient to the operating room?) and planning interventions (is the secondary insult from an orthopedic procedure likely to worsen cardiopulmonary physiology?).
Pathophysiology of Pulmonary Dysfunction
Multiply injured patients are at risk for major pulmonary dysfunction because of disruption of three key elements. First, brain injury is common in patients with chest injuries, resulting in inadequate respiratory drive, or inability to maintain patent proximal airways. Second, injury to the torso can produce changes in compliance, ineffective respiratory effort, and pain that impact the patient’s ability to complete the work of breathing. Third, insults to the lungs themselves result in ineffective gas exchange and hypoxemia. In polytrauma patients, it is likely the clinician must consider simultaneous insults affecting all three elements. Impaired airway patency (e.g., diminished level of consciousness), increased work of breathing (e.g., multiple rib fractures), and impaired gas exchange (e.g., pulmonary contusion, fat emboli syndrome) often coexist.
At the same time that these patients experience impaired gas exchange, they actually have a marked increase in respiratory demand because the neurohormonal response to injury results in increased cellular metabolism. This creates a substantial increase in CO2 production that must be matched by increased elimination from the lungs. While a resting adult eliminates 200 cc/kg/min of CO2, postinjury hypermetabolism results in CO2 production in the range of 425 cc/kg/min [7]. Thus, the minute ventilation required to maintain a normal pH may rise from a resting rate of approximately 5 L/min to more than 10 L/min. This represents a 100 % increase in ventilation simply to meet metabolic demands. To make matters worse, injured patients typically have an increase in physiologic and anatomic pulmonary dead space—ventilated regions of the lung that do not participate in gas exchange. In a normal adult, the proportion of each breath that is dead space (Vd/Vt) is approximately 0.35. For injured patients with pulmonary failure, the Vd/Vt often exceeds 0.6. Simply put, extra dead space means each breath is less effective at eliminating CO2. Therefore minute ventilation requirements in the 12–20 L/min range are not uncommon in the postinjury setting.
In this light, secondary insults that further impair gas exchange or further increase metabolic rate may cause a stable patient to decompensate; as discussed below, orthopedic interventions are uniformly associated with worsening gas exchange. This makes timing of bony fixation a challenging puzzle. If we do not definitively repair fractures, we impair the respiratory system by immobilizing the patient (impaired work of breathing, increasing dead space, ineffective cough). Alternatively, If we opt for definitive fixation in a tenuous patient, we may impair the pulmonary system by worsening gas exchange and increasing metabolic demand.
The patient with chest injuries faces hurdles in meeting increased respiratory demand. Respiratory drive may be impaired by brain injury and by medications routinely used for sedation and analgesia. The energy required to complete a respiratory cycle is increased by chest wall edema and recumbent positioning, which is often prolonged in patients with major bony injuries. Muscular weakness from impaired energetics (acidosis, cardiovascular failure, mitochondrial dysfunction, oxidant stress) or fatigue may be an insurmountable challenge. Decreased pulmonary compliance from an increase in extravascular lung water and pleural collections (effusions/hemothorax) also contributes. Lastly, and perhaps most importantly, pain from torso injuries or operative interventions make the increased ventilatory demand a substantial burden to the patient.
Primary Injury Patterns
Rib Fractures and Flail Chest
Rib fractures are the most commonly identified chest injury in the multiply injured patient. Crude rates of morbidity and mortality are consistently associated with the number of broken ribs, particularly in elderly patients [8, 9]. Patients with multiple rib fractures are thought to be at high risk for pulmonary failure and pneumonia—likely from impaired cough, atelectasis from splinting, and inability to execute the work of breathing if pain control is poor. A recently published analysis of over 40,000 patients queries this association a bit more closely [10]. This work by Jones et al. highlights that in patients whose only injuries are rib fractures, mortality is less than 6 %. Further, when early (<24 h) deaths are excluded, crude mortality, while still related to number of fractures, is less than 10 % across all groups. The most powerful predictor of mortality was the abbreviated injury score for the chest region, reflecting the potential importance of flail chest (below) and injuries involving the pleural space, lung, and mediastinal structures. The theme again here is that patients who surviving long enough to warrant orthopedic interventions are unlikely to die from their thoracic injury, and thus minimizing secondary insults becomes pivotal in achieving excellent outcomes.
Flail chest is a pattern of injury wherein a portion of the chest wall loses bony continuity with the rest of the respiratory pump. This most commonly occurs when multiple adjacent ribs are fractured in more than one location. It can also occur in association with sternal fractures or disruptions of costochondral junctions. When the patient expands their chest to take a breath, creating negative intrathoracic pressure, the disconnected area (“flail segment”) moves inward in a paradoxical fashion. Particularly when this injury occurs in concert with a major loss of thoracic volume (“caved in chest”), the expansion of the underlying lung is attenuated, and there may be decreased effective tidal volume and therefore impaired ventilation.
Acutely, flail chest injuries per se are not frequently an early threat to life, with mortality reported as less than 10 % in modern series [11, 12]. In isolated flail chest, for example, most patients will not need mechanical ventilation. The major initial challenge is pain control and pulmonary hygiene as ineffective cough and ability to execute increased ventilatory demands are common. In long-term follow-up [13], this is a morbid injury pattern to be sure, as chronic pain, chronic dyspnea, and disability are a common outcome. The significance of a flail chest in the acute setting largely relates to the fact that it denotes major energy transfer to the thorax. This is particularly true in younger patients, where ribs are relatively elastic—more likely to transiently deform than to fracture. Major bony injuries to the chest wall in a young patient with a major mechanism of injury (e.g., high-velocity motor vehicle crash) signify a high likelihood of underlying pulmonary contusion (see below) and extrathoracic injuries [14].
Supportive care remains the mainstay of treatment in rib injuries, with or without flail chest, though there is renewed and justified interest in rib fixation. Acute mortality for chest wall injury is low, but long-term morbidity is substantial and is largely related to malunion—which would appear eminently preventable. Design of rib-specific hardware permits a more practical approach, and newer techniques involving plates with some elasticity as well as minimally invasive approaches may continue to fuel enthusiasm for operative treatment. A number of small published series suggest improved short-term outcomes [15, 16]. The current challenge is identifying patients who are likely to substantially benefit. For example, the patient with other major injuries that may result in prolonged ventilator dependence (brain injury, open abdomen, spinal cord injury) may not benefit acutely or long term from chest wall fixation.
Pulmonary Contusion
Pulmonary contusion, simply put, is a bruise of the lung. The most common presentation is a young passenger struck on the nearside compartment; rapid deceleration and frontal crashes into fixed objects are frequent contributors [17]. Three different types of forces combine to produce injury to the lung. First, direct transmission of energy through the chest wall can bruise the lung. Secondly, the lung can be bruised by shearing forces, for example, when a high-energy missile passes through the lung parenchyma, there is a zone of contusion around the tract of the missile. Thirdly, blast or concussive injury can produce significant lung contusion without obvious chest wall damage. An isolated pulmonary contusion is pathologically similar to bruises elsewhere. The initial response is edema and hemorrhage. This is followed by inflammation, recruitment of cellular elements to the zone of injury, and then by repair. The clinical course follows a similar pattern. As the swelling and inflammation evolves, there is worsening of pulmonary compliance and gas exchange. This continues for 48–72 h after which improvement should be expected. Some mild hemoptysis can be expected as hemorrhagic secretions are cleared from the distal airways.
An initial chest x-ray is diagnostic in patients with large contusions. Smaller injuries may not become evident until later—when swelling and inflammation occur. Approximately one third of patients with blunt chest injuries will have evidence of pulmonary contusion on Computed Tomography (CT) that was not appreciated on initial plain radiographs [18]. CT has thus been promulgated as a more sensitive tool for diagnosis, and a number of scoring systems have been developed. Strumwasser et al. [19] analyzed 106 consecutive patients undergoing CT of the chest for blunt multitrauma. They observed that a computed tomography volume index (estimating the fraction of total lung involved by contusion) was an independent predictor of ICU length of stay. Additionally, pts with a CT volume index >0.2 had, on aggregate, a higher risk of pneumonia, ARDS, and death. In a larger retrospective series from Boston (almost 400 pts), a score of 1–6 was used, based on presence or absence of contusion in three zones of each lung [20]. They observed that mechanical ventilation was required more often in patients with a score >2, and this was an independent risk factor for the need for ventilation (odds ratio = 13); this can be thought of as 50 % or more of lung zones involved with contusion. That being said, only 35 % of patients with BPC6 >2 required mechanical ventilation. Additional factors also predictive of mechanical ventilation included diminished Glasgow Coma Scale (GCS) score and >4 rib fractures. Wang et al. observed that PC volume predicted chest trauma patients who would later meet criteria for adult respiratory distress syndrome [21].
It would seem logical, given these studies, that patients with large pulmonary contusions evident on radiographs should be recognized as at increased risk for secondary pulmonary insults. A different and as yet unanswered question is whether broad application of CT scanning for blunt chest injuries is cost effective for pulmonary contusion, as management is entirely expectant and treatment entirely supportive. Some studies strongly suggest that contusions identified only on CT scanning are of limited clinical significance [22]. Further, areas of dependent edema, consolidation, or aspiration pneumonitis may be mistaken for contusion. Certainly, if imaging is already done and available, it should be used to guide decision making, but in the tenuous patient, a trip to CT scan may represent an unnecessary risk.
Early evolution of the patient’s gas exchange must be taken into account. Patients with early (<6 h) impairment in oxygenation should be approached with caution. The most common tool for describing impairment in oxygenation is the ratio of arterial partial pressure of oxygen (paO2—the tension of oxygen in the blood) to the percentage (fraction) of oxygen the patient is inhaling (FiO2—how much oxygen is the patient on). This is commonly referred to as the P/F ratio. At sea level, a normal P/F is about 400. Impairment in gas exchange results in progressively lower values, with mild impairment being <300, moderate <200, and severe <100.
Blunt Cardiovascular Injuries
Like the lung, the heart may be bruised by direct, shear, or blast forces. Since the true “gold standard” for myocardial contusion would be direct examination or biopsy, it is difficult to assess any particular diagnostic approach for sensitivity and specificity. Thus, for practical purposes, one should consider that there are only two common sequelae of blunt cardiac injury: arrhythmia and pump failure. Many of the arrhythmias associated with blunt trauma are relatively benign (sinus tachycardia, atrial fibrillation), and an initial EKG that is normal is associated with a very low chance of a malignant arrhythmia [23]. Thus, an early EKG can be advocated to identify patients at risk. With respect to pump failure, the most common cause is a major contusion of the right ventricle (which lies more anterior), and this typically presents early as hypotension refractory to volume replacement. In these rare cases, early echocardiography can be recommended to confirm the diagnosis. In patients without clinical evidence of pump failure, the utility and clinical significance of cardiac enzyme measurement, while advocated by some, is a matter of some debate.
Other cardiovascular injuries such as great vessel injury, pericardial rupture, and cardiac rupture are remarkably rare and are largely beyond the scope of this chapter. One injury worth mentioning both in terms of incidence and significance is the torn descending thoracic aorta. While historically described as an immediate threat to life, many of these injuries can be safely observed in the stable patient, and intervention planned for a time when the patient is physiologically well enough to sustain an additional insult [24–26]. Minimally invasive approaches using stent grafts are rapidly replacing operative repair, though long-term follow-up is far from complete. There are some concerns about the ultimate fate of stent grafts placed in young patients, as graft collapse and migration have been described, and it is unclear what will evolve as the young aorta gradually dilates with age and a fixed stent graft remains in place. With respect to operative repair of the torn aorta, many of the risk factors that make any secondary procedure potentially unwise apply: major pulmonary contusion, poor gas exchange, and injuries that would make anticoagulation contraindicated.
Secondary Pulmonary Injury
Acute Respiratory Distress Syndrome (ARDS)
The syndrome of ARDS was outlined in a small series of patients by Ashbaugh and Petty in 1967 [27], and the essence of this description remains today. The main components in the clinical setting include (1) hypoxemia refractory to oxygen administration; (2) diffuse, bilateral infiltrates on imaging of the lungs; and (3) decreased lung compliance. A standard definition of ARDS has been in use for almost 20 years. The Consensus Conference of North American and European investigators (NAECC) agreed that ARDS should be viewed as the most severe end of a spectrum of an acute lung injury (ALI) [28]. The diagnostic criteria for ARDS include acute onset, the PaO2/FiO2 200 mmHg or less (<300 for ALI), bilateral infiltrates on chest radiograph, and no evidence of left atrial hypertension (either clinical or with direct measurement). During the last two decades, some limitations of this definition have been apparent, including an unclear meaning of “acute,” the unclear role of transient changes in the P/F ratio in establishing the diagnosis, and potential inclusion of a broad array of patients with hypoxemia. Additionally, recruitment of collapsed lung tissue (predominantly with positive end-expiratory pressure, PEEP) may result in a remarkable improvement in P/F ratio in a short period of time—does this patient no longer have the syndrome ARDS? Lastly, while the NAECC definition excludes patients with left atrial pressure (LAP) >18, Ferguson et al. [29] showed that patients with no risk factors for congestive heart failure but with a clinical syndrome of ARDS commonly had LAP >18.
Recently proposed changes to the ARDS definition have been developed using consensus methodology in a series of meetings in Germany. This new “Berlin definition” of ARDS is likely to be widely embraced and offers significant advantages over the 1994 definition [30]. In particular, it defines “acute” more precisely, drops the term ALI (which may be confused as a separate entity), and provides for a larger consideration of precipitating factors when CHF and ARDS may coexist. This modern definition of ARDS is shown in Table 14.3.
Table 14.3
Summary of the proposed “Berlin” definition of ARDS
Factor | Description |
---|---|
Onset | Within 1 week of known risk factor |
Imaging | Bilateral opacities not explained by effusion, collapse, or nodules |
Type of pulmonary edema | Not explained by cardiac failure or fluid overload. If no clinical risk factor identified, objective assessment required |
Severity (with PEEP≥5) | Mild: P/F ≤ 300 |
Moderate: P/F ≤ 200 | |
Severe: P/F ≤ 100 |
Clinical risk factors for ARDS can be broadly categorized into direct and indirect groups. Direct factors are those primarily associated with local pulmonary parenchymal injury and include pulmonary contusion, aspiration, and pulmonary infection. Indirect factors are those thought to be associated with systemic inflammation and resultant lung injury. These include severe sepsis, transfusion of banked red cells, transfusion of FFP, and multiple long bone fractures [31]. Unless shock is associated with significant tissue injury or other known risk factors (e.g., transfusion), it is generally not known to precipitate ARDS. Orthopedic injuries are consistently found to be an independent risk factor for ARDS, particularly in the case of femur fractures [32–35]. Commonly observed risk factors for ARDS are shown in Table 14.4.
Table 14.4
Commonly observed risk factors for the adult respiratory distress syndrome
Direct | Indirect |
---|---|
Pulmonary contusion | Severe sepsis |
Aspiration | Severe trauma |
Pneumonia | Pancreatitis |
Pulmonary ischemia/reperfusion | Extrapulmonary (e.g., splanchnic) Ischemia/reperfusion |
Fat emboli syndrome | Transfusion |
We currently understand ARDS as an immuno-inflammatory injury to lung tissue which produces markedly impaired gas exchange [36]. This paradigm posits that both infectious and noninfectious insults initiate a generalized inflammatory response that subsequently injures the lung in an autotoxic fashion. Mediators proposed to initiate this response include danger-associated molecular patterns (DAMPs, released from soft tissue injury), leukocytes/lipid/protein mediators from stored blood components and leukotrienes elaborated from gut lymph. Most relevant to the current discussion is the observation that several components of bone marrow and fracture serum can initiate or exacerbate this phenomenon, including particulate matter, arachidonic acid metabolites, and proinflammatory cytokines [34, 37, 38]. These mediators can initiate indiscriminate activation of effector cells (predominantly macrophages and neutrophils) that subsequently release oxidants, proteinases, and other factors that promote tissue injury.
If the initial insult is severe enough, early organ dysfunction results (“one-hit” or single insult model). More often, a less severe insult results in a systemic inflammatory response syndrome (SIRS) that may not be injurious. These patients appear, however, to be “primed” such that they have an exaggerated response to a second insult, which leads to an augmented/amplified systemic inflammatory response and multiple-organ dysfunction [39]. Fixation of fractures, which may represent additional soft tissue injury, blood loss, and release of mediators from bone marrow/fracture sites, is often thought to be a second insult. In that light, understanding which patients are at risk for ARDS from insults which can be planned may be pivotal in the care of the multiply injured patient.
The characteristic lesion of ARDS affects the interface between alveoli and pulmonary capillaries, with both epithelial and endothelial damage, resulting in a high permeability pulmonary edema. Changes in this lesion—and in the patient’s physiology—follow a typical pattern, usually divided into three overlapping phases: (1) the exudative phase, with edema and hemorrhage; (2) the proliferative phase, with organization and repair; and (3) the fibrotic phase [40]. The exudative phase is apparent in the first 3–7 days. Histologic changes include proteinaceous alveolar edema, interstitial edema, and intra-alveolar hemorrhage. The exudative phase is characterized by the appearance of hyaline membranes, which are composed of cellular debris and plasma proteins. Loss of the alveolar epithelial barrier results in alveolar edema, as the remaining cells are unable to drive sodium from the alveolar into the interstitial compartment.
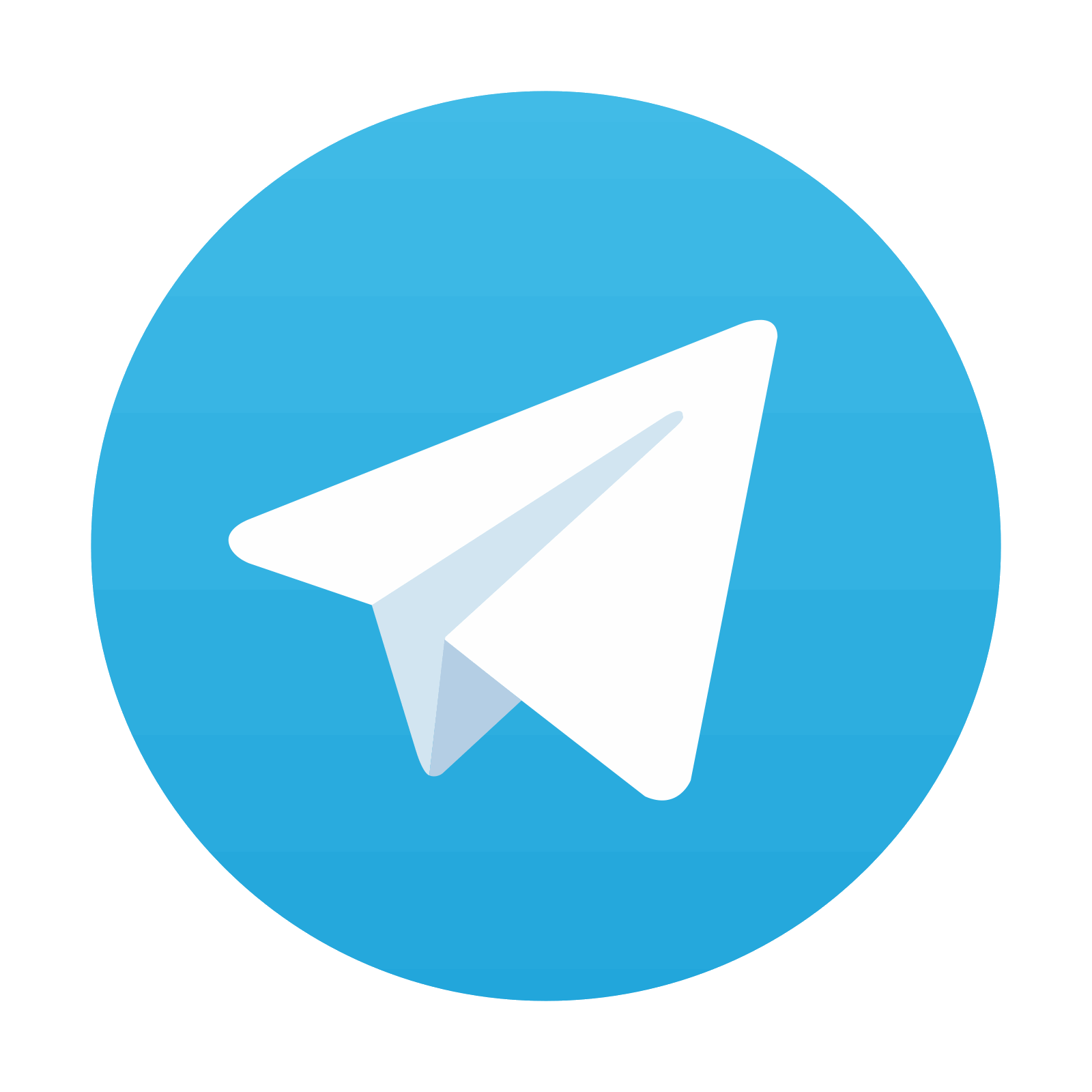
Stay updated, free articles. Join our Telegram channel
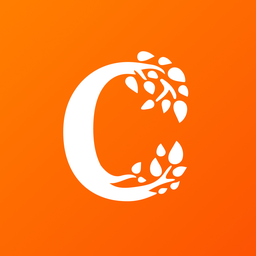
Full access? Get Clinical Tree
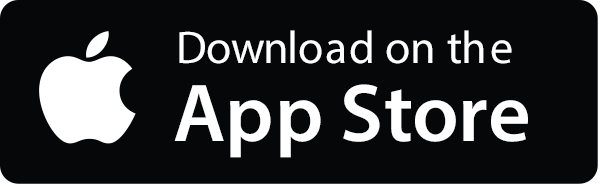
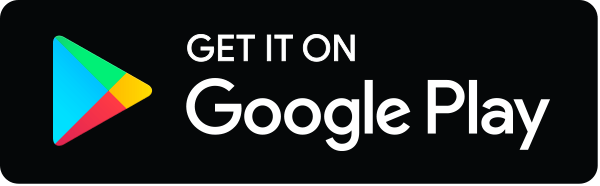