material and then reimplanted into a focal defect in the same joint. The recent approval of the matrix-assisted autologous chondrocyte implantation (MACI) procedure represents the first “tissue engineered” produce in clinical use in the musculoskeletal system.2
TABLE 1 Cell Types for Musculoskeletal Regeneration and Repair | ||||||||||||||||||||
---|---|---|---|---|---|---|---|---|---|---|---|---|---|---|---|---|---|---|---|---|
|
one-stage procedures.9 Finally, with this and other approaches, methods are being developed to select the most efficient cartilage forming cells, including from alternative sources (such as nasal cartilage),10 as well as to remove from the population those showing senescent or other aberrant phenotypes.11
be engineered to express a number of factors, including those that promote their own differentiation or factors that improve the host environment to increase retention/survivability after implantation.30 This could improve their functional role, but it should be noted that any cell type that is more than “minimally manipulated” will face a more stringent review by the FDA prior to approval for clinical use.
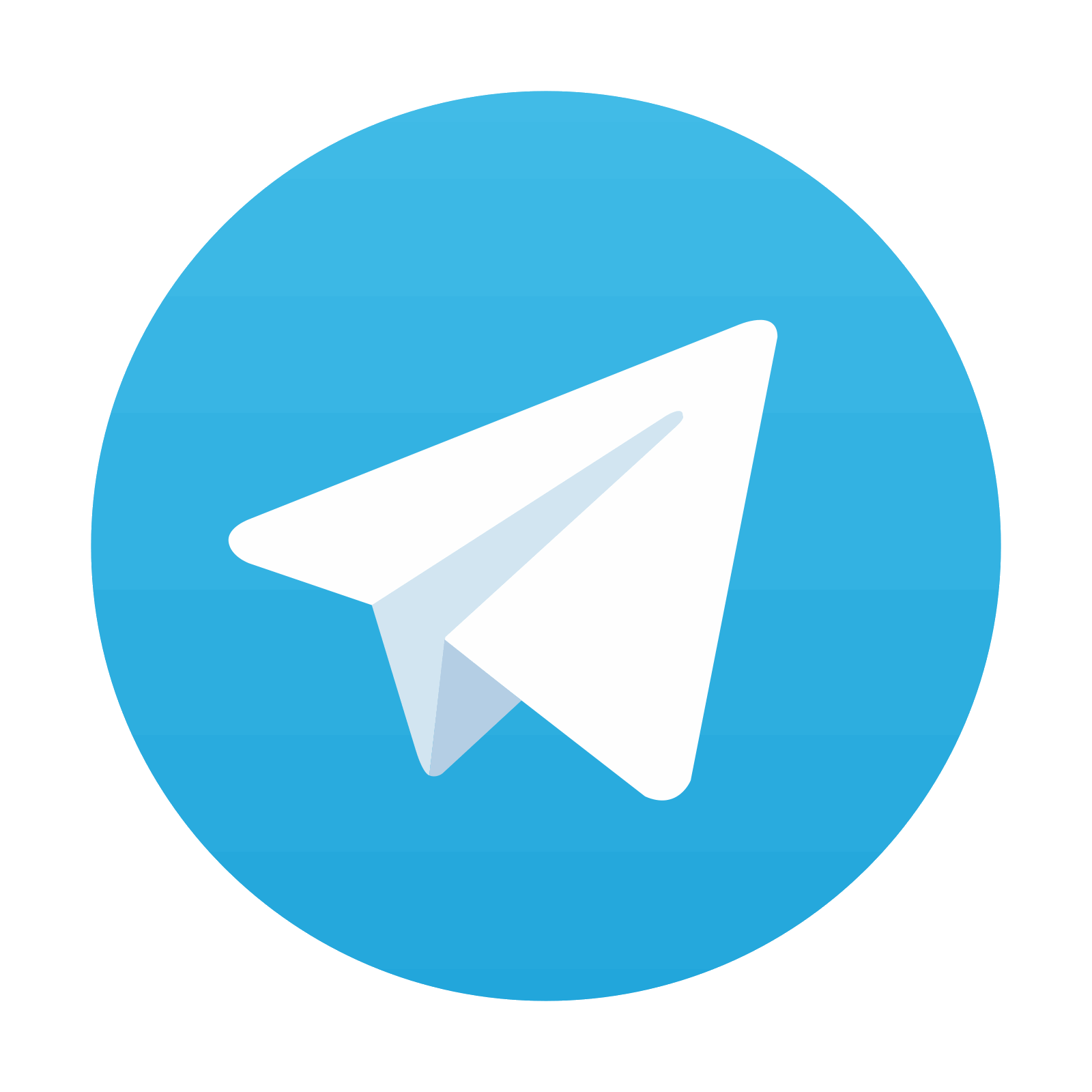
Stay updated, free articles. Join our Telegram channel
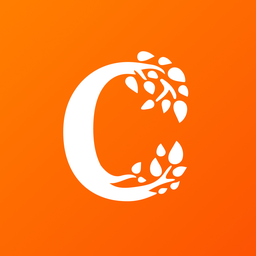
Full access? Get Clinical Tree
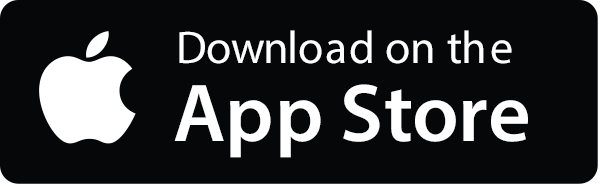
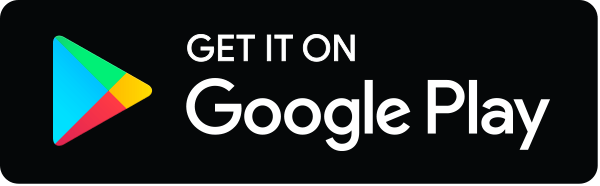
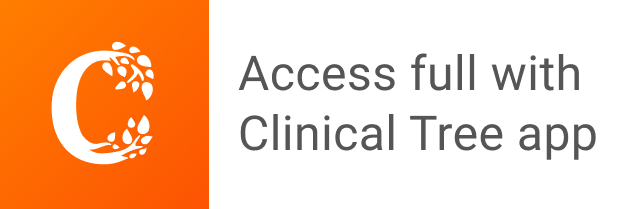