Fig. 5.1
Arthroscopic image of microfracture
Microfracture is a technique introduced by Steadman et al., which involves the accurate debridement of all unstable and damaged articular cartilage, down to the subchondral bone plate while maintaining a stable perpendicular edge of healthy cartilage [16]. An arthroscopic awl is used to make multiple holes in the defect 3–4 mm apart, ensuring the subchondral plate is kept intact. The defect is filled with so-called super clot, which is purported to be the optimal environment for pluripotential marrow cells to differentiate into stable tissue (Fig. 5.1) [16]. The rehabilitation protocol is an important part of Steadman’s procedure. Early mobility of the joint with continuous passive motion is advocated in conjunction with reduced weight bearing for an extended period.
These techniques are simple and minimally invasive. They can be performed arthroscopically and do not require complex equipment or instrumentation. They are readily available and inexpensive. The advantages of microfracture over drilling might include reduced thermal damage to subchondral bone and the creation of a rougher surface to which repair tissue might adhere more easily. It is also easier to penetrate a defect perpendicularly with a curved awl during an arthroscopic procedure as compared with a drill.
5.2.1.2 Limitations
While often the simplest option for small isolated defects, fibrocartilage is mechanically inferior to hyaline cartilage (composed of type II collagen) [19]. It has also been noted that there is an unpredictable volume of cartilage repair with microfracture [20]. In addition, the failure rates for subsequent cell transplantation are greater in patients that had a prior microfracture. Marrow stimulation techniques have, therefore, been considered by some to be merely a pain-relieving procedure that at best delays the progression towards osteoarthritis [7, 11].
5.2.1.3 Complications
It has been reported that microfracture can have a significant effect on the micro- and macro-architecture of subchondral bone [18]. A number of authors have reported subchondral cysts and intralesional osteophytes from 6 months to 5 years postoperatively [21–23]. Recently, it has been postulated that subchondral cyst formation is caused by infiltration of cytokines and metalloproteinases into the subchondral bone subsequent to microfracture [18]. This may explain why the outcome of subsequent cartilage procedures following microfracture has been reported as suboptimal [24].
5.2.1.4 Biological Augmentation of Microfracture
There is increasing evidence that modification or augmentation of microfracture may have the potential to enhance the quality of the repair tissue formed over the cartilage defect and the prospect for improved clinical outcomes [25, 26]. The techniques described seek to provide a biological augment to the microfracture site by delivering cells (i.e. stem cells) and/or individual growth factors (i.e. platelet-rich plasma), with or without the addition of a scaffold material [27–29]. For example, BST-CarGel, a chitosan-based medical device, which is mixed with autologous whole blood and applied to a microfractured cartilage lesion, is thought to physically stabilise the clot and guides and enhances marrow-derived repair [30]; the results of a randomised control trial comparing this treatment to microfracture are discussed later on in the chapter.
5.2.2 Cellular Regeneration Techniques
5.2.2.1 Description of Technique
Cell-based options are used in an attempt to repair hyaline cartilage defects with chondrocyte or stem cell implantation. Autologous chondrocyte implantation (ACI), one of the first applications of cell engineering in orthopaedics, was first performed by Peterson et al. in Gothenburg in 1987 [31]. Cartilage is harvested at an initial arthroscopy, and culture-expanded autologous chondrocyte cells are injected into a chondral defect underneath a patch of periosteum or collagen membrane (Fig. 5.2a–c) [32].
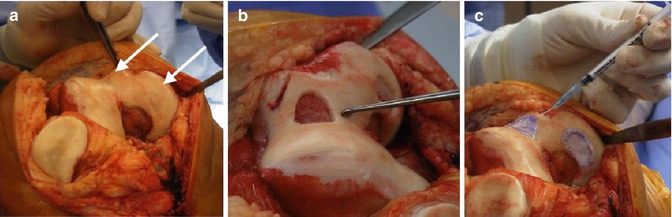
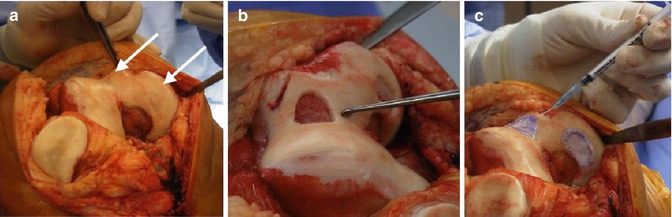
Fig. 5.2
(a–c) Operative images of ACI performed to treat cartilage lesions of the trochlea and medial femoral condyle of the right knee: (a) two separate full-thickness cartilage lesions (white arrows), (b) preparation of the defect to the subchondral plate with well-defined edges and (c) injection of chondrocytes beneath a collagen patch (Images provided courtesy of Professor David Wood – University of Western Australia)
In studies where histological analysis has been performed, it is reported that ACI is capable of producing tissue, which is hyaline-like in some specimens [33]. A variation of the ACI technique using culture-expanded bone marrow stem cells has the advantage of not requiring an additional arthroscopic procedure in order to harvest articular cartilage which has also reported good results [34]. In an attempt to reduce the dedifferentiation of cultured chondrocytes, characterised chondrocyte implantation (CCI) was developed [35]. This technique selects cells with a stable chondrocyte phenotype, which is thought to produce a better quality fill of the defect and enhanced biomechanical properties [35, 36].
Matrix-assisted autologous chondrocyte implantation and related techniques are regarded as second-generation forms of cell implantation that provide a three-dimensional structure for cell adhesion, proliferation and matrix production [37]. A biodegradable type I/III collagen membrane provides a scaffold for cultured autologous chondrocytes, which are seeded onto the surface (Fig. 5.3a–c) [38]. Implantation may be performed arthroscopically or via mini-arthrotomy [39]. Scaffolds are also being used with undifferentiated cell sources, like mesenchymal stem cells derived from bone marrow, synovium and other sources suitable for insertion in a single-stage operative procedure [40–42].
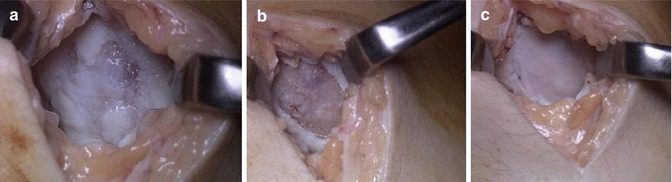
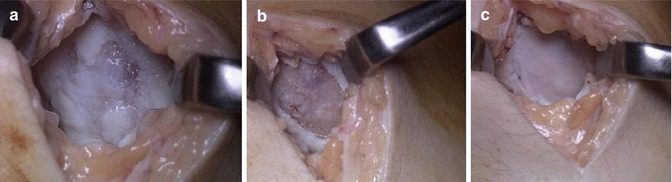
Fig. 5.3
(a–c) Operative images of MACI to treat a cartilage lesion on the medial femoral condyle of the left knee: (a) medial arthrotomy of the knee demonstrating a full-thickness ulcerated cartilage lesion, (b) debridement of the lesion to the subchondral plate and (c) insertion of the synthetic matrix to fill the defect (Images provided courtesy of Professor Julian Feller – OrthoSport Victoria)
5.2.2.2 Limitations
Although more similar to hyaline cartilage, the best repair tissue achieved as a result of ACI is still not morphologically or histochemically identical to normal hyaline cartilage, and fibrocartilage may be found in a proportion of samples [33]. Whereas some studies have reported that prior bone marrow stimulation and opposing chondral lesions lead to a higher risk of failure, others have shown satisfactory outcomes in both these patient groups [43–46]. Periosteal patch hypertrophy was a significant concern in first-generation ACI, but subsequent generations with enhanced membrane materials have reduced this risk [17]. One of the major drawbacks with ACI, however, is that implantation requires two separate operative procedures with an intervening period of cell culture. This not only creates substantial cost and inconvenience at a clinical level but also adds to the propensity for chondrocytes to dedifferentiate towards a fibroblastic phenotype during culture [47]. Although the literature suggests that procedures using three-dimensional scaffolds are safe, both matrix-assisted autologous chondrocyte implantation and alternative cell-scaffold techniques are still only available for use outside the USA because of variations in their regional regulation [12].
5.2.2.3 Complications
A major proportion of complications after ACI can be summarised by four major diagnoses: symptomatic hypertrophy, disturbed fusion, delamination and graft failure [48]. Among those, the overall complication rate and incidence of hypertrophy of the transplant were higher for periosteal ACI [48]. A systematic review by Harris et al. determined that the failure rate is highest with periosteal ACI and lower with collagen-membrane cover ACI and second-generation techniques [49]. One third of ACI patients underwent a reoperation. Unplanned reoperations were most commonly seen following periosteal ACI, where hypertrophy and delamination were the most frequent complication. Arthrofibrosis was most commonly seen after arthrotomy-based ACI. The use of second-generation techniques and all-arthroscopic techniques reduced the failure, complication and reoperation rate after ACI [49].
5.2.3 Chondral and Osteochondral Transplantation
5.2.3.1 Description of Technique
Osteochondral autologous transplantation (OAT) is a technique that aims to replace the articular cartilage defect with hyaline articular cartilage plugs harvested from elsewhere in the knee (Fig. 5.4) [50]. Osteochondral autograft plugs and mosaicplasty (multiple osteochondral autograft plugs of a smaller diameter) are used to provide a whole osteochondral unit. The advantage of this method of treatment relates to the ability of autogenous bone to integrate more readily compared to cartilage, thus preserving the cartilage-bone interface [51].
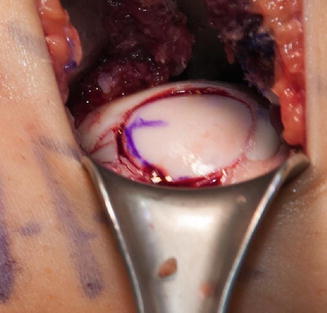
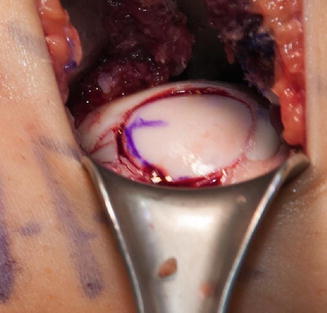
Fig. 5.4
Press-fit allograft using OAT technique to restore the articular congruency of the posterior femoral condyle
Fresh osteochondral allografts may also be used. They are particularly useful for the treatment of larger chondral defects, especially when there is damage to the underlying bone, e.g. cyst formation [52]. Osteochondral allografts are often used for salvage of failed prior cartilage procedures. Off-the-shelf natural or synthetic osteochondral scaffolds that can be impregnated with suitable cells may also be used [53].
Chondral graft is an alternative treatment method, which has come to prominence recently. Minced cartilage autograft and particulated juvenile cartilage allograft have been reported as grafts for chondral repair [54–56]. Histological analysis of both techniques has demonstrated that transplanted cartilage cells migrate from the extracellular matrix, proliferate and form a new hyaline-like cartilage tissue matrix that integrates with the surrounding host tissue. The techniques for minced or particulated grafts are performed in a single-stage procedure and are relatively straightforward. Short-term results have demonstrated that the procedures are safe and effective [56, 57].
5.2.3.2 Limitations
Graft-site mismatch is a potential limitation of osteochondral autograft [58]; grafts may be of different thickness or rotational orientation than the host site and may not be perfectly flush or parallel. Using the mosaicplasty technique may result in gaps. Whereas the bone component of the graft heals, there is no side-to-side healing of the articular layer between the graft and the host. The difference in cartilage thickness and, therefore, mechanical properties of the graft remains a concern [58]. As a result the native biomechanics of the joint may not be recreated. Autograft is also not always feasible for the treatment of large lesions due to the potential for donor site morbidity.
As regards osteochondral allograft, poorer results have been found in older patients, bipolar and patellofemoral lesions and corticosteroid-induced osteonecrosis [59]. Mismatch is also a concern with large allograft, particularly related to the size and depth of the graft and also the contour of the articular surface. Allogenic tissue also has the potential for disease transmission. There is also questionable chondrocyte viability and a lack of integration with surrounding tissue.
The experience with chondral grafting is limited, and given the long-standing belief that integration requires osseous contact, the long-term survival and integration of the graft with host tissue requires further study. Synthetic osteochondral plugs are a very attractive treatment option; however, the stringent regulatory processes required for approval of this technology are extremely challenging not to mention costly. It has been estimated that it may cost up to $500 million to bring a new biological option to the market in the USA [12].
5.2.3.3 Complications
Despite the maintenance of the integrity of osteochondral autograft plug following transfer, the surround articular cartilage can continue to deteriorate, leading to a wide area of further chondral damage [50]. Graft delamination has been reported with osteochondral grafting and also with particulated cartilage grafting [54, 60]. Articular surface incongruity and failure of osseous incorporation can also occur following osteochondral grafting, which is best appreciated on postoperative MRI [60]. Osteochondral allografts are also capable of causing kissing lesions on the tibial plateau if left too proud on the femoral condyle [61]. Recent studies on a biphasic synthetic plug have presented concerning findings with respect to both clinical outcomes and structural analysis, with the finding of fibrous tissue repair and foreign-body giant cells at the defect site at the time of revision surgery [53].
5.3 Evidence-Based Practice
Despite an increase in research focus on the treatment techniques available for articular cartilage defects of the knee, there remains no consensus as to the best available treatment option [62]. Furthermore, the vast majority of these studies have been of low methodological quality; only 9 % of 194 studies on cartilage treatment were level I randomised controlled trials [63] with the majority (76 %) being level IV evidence.
For the purpose of providing clarity on the best available evidence in the literature, a review of the systematic reviews is provided in this chapter along with an up-to-date systematic review of all level I studies performed to assess the outcome of a variety of cartilage repair and regeneration techniques.
5.3.1 Review of Systematic Reviews
Eighteen systematic reviews have been identified which focus on a variety of articular cartilage treatment techniques (Table 5.1). As with any systematic review, the evidence provided is only as good as the original studies chosen as part of the selection process. Only two studies provided a review of level I evidence [72, 76]. Vasiliadis et al., in their study, determined that there was insufficient evidence available from level I studies to support ACI over OAT, MACI or microfracture. Moreover, Bekker et al. could only identify four level I evidence studies published between 2003 and 2008 [76]. The authors concluded that smaller lesions should be treated by microfracture or single plug OAT and that ACI or OAT resulted in improved outcomes in active patients with large articular cartilage lesions compared to microfracture.
Table 5.1
A review of systematic reviews
Author | Year | Number of studies | Systematic review | Comparative treatments | Level of evidence | Clinical outcome |
---|---|---|---|---|---|---|
De Caro et al. [64] | 2015 | 11 | Fresh osteochondral allograft | Level IV | Good long-term result with fresh osteochondral allograft. Procedure burdened by cost and access to grafts | |
Kon et al. [65] | 2015 | 127 | Scaffolds with and without cells | Clinical setting – cells and no cells | Levels I–IV | Good short-term outcome with scaffolds with and without cells |
Lynch et al. [58] | 2015 | 9 | OAT | MF, ACI | Levels I–II | OAT effective in smaller lesions (<2 cm2). Known risk of failure at 2–4 years |
Oussedik et al. [66] | 2015 | 34 | MF or ACI | CCI, MACI | Levels I–IV | MF effective in smaller lesions, MACI more effective than MF, ACI an effective treatment |
Goyal et al. [67] | 2013 | 15 | MF | ACI, CCI, OAT | Levels I–II | MF had good clinical outcomes in small lesions and lower-demand patients. Treatment failures identified at 5–10 years |
Filardo et al. [68] | 2013 | 18 | MSC | Levels III–IV | Insufficient evidence | |
Filardo et al. [62] | 2013 | 51 | Scaffold-based repair | Levels I–IV | Insufficient evidence | |
De Windt et al. [69] | 2013 | 9 | All treatments in the setting of early OA | ACI, CF, MF | Level IV | ACI could provide good short- to medium-term clinical outcomes in the setting of early OA |
Chahal et al. [70] | 2013 | 14 | OAT | Level IV | Favourable outcomes and high satisfaction rates at intermediate follow-up | |
Vavken et al. [71] | 2010 | 9 | ACI | MF, OAT | Levels I–II | Insufficient evidence |
Vasiliadis et al. [72] | 2010 | 6 | ACI | OAT, MACI, MF | Level I | Insufficient evidence |
Harris et al. [73] | 2010 | 13 | ACI | OAT, MF | Levels I–II | ACI associated with improved outcomes in defects >4 cm2. ACI and OAT gave comparable short-term results |
Nakamura et al. [74] | 2009 | 39 | Cell-based therapy | ACI, CCI, MACI | Levels I–IV | Insufficient evidence |
Mithoefer et al. [20] | 2009 | 28 | MF | Levels I–IV | MF has effective short-term outcomes | |
Kon et al. [75] | 2009 | 18 | MACI | Levels I–IV | Insufficient evidence | |
Bekkers et al. [76] | 2009 | 4 | All treatments | ACI, CCI, MF, OAT | Level I | MF had good results with <2.5 cm2 lesions, ACI or OAT superior if lesion >2.5 cm2. Active patients have better results with OAT or ACI |
Magnussen et al. [77] | 2008 | 6 | All treatments | ACI, MACI, MF, OAT | Levels I–II | No technique was superior |
Ruano-Ravina and Diaz [78] | 2006 | 9 | ACI | MF, OAT | Levels I–IV | No evidence of superiority of ACI |
A further five systematic reviews contain both level I and level II evidence. Of these systematic reviews, two found that there was insufficient evidence to determine a superior treatment between ACI, OAT and microfracture [71, 77]. The remaining three studies provided differing conclusions; Lynch et al., comparing OAT with microfracture and ACI, concluded that OAT is effective in smaller lesions (<2 cm2), but ACI has superior long-term results, albeit the latter conclusion was based on the results of a single randomised control conducted by Bentley et al. in 2012 [58, 79]; Goyal et al., in their comparison of microfracture versus CCI, ACI and OAT, reported that microfracture has good clinical outcomes in small lesions and lower-demand patients; however, treatment failures were identified at 5–10 years [67]; finally, Harris et al., comparing ACI with OAT and microfracture, stated that ACI was associated with improved outcomes in defects >4 cm2 and ACI and OAT gave comparable short-term results [80]. Interestingly, these studies were all carried out over a period of 5 years, which emphasises the need to be cautious in reading too much into systematic reviews as very similar data can be interpreted quite differently.
Thirteen further systematic reviews were analysed. Three studies contained a review of only level IV evidence [64, 69, 70]. In two of these three studies, only one technique was studied, and unsurprisingly, the results demonstrated favourable outcomes. The third study by Windt et al. focused on all cartilage treatment techniques in the setting of early osteoarthritis and determined that ACI could provide good short- to medium-term outcomes. Of the remaining eight articles, the level of evidence ranged from level I to IV; six out of these eight studies determined that there was insufficient evidence to determine superiority for any of techniques assessed, thus providing little clarity on the dilemma of which articular cartilage treatment to use.
5.3.2 Level I Evidence: An Up-to-Date Systematic Review
For the purpose of this chapter, an up-to-date systematic review was performed of only level I studies according to PRISMA guidelines (Fig. 5.5). The studies included in this systematic review were all randomised controlled trials of a variety of articular treatment techniques, representing the most widely accepted practices internationally, with both short- and long-term results being reported. Studies were only selected if they met exacting methodological criteria and had a low risk of bias. Nine studies were identified with the highest level of clinical evidence from the current body of research on the surgical treatment of articular cartilage lesions of the knee (Table 5.2). The main finding was that regardless of the type of cartilage regeneration technique used, an improvement in the measured clinical outcome was observed compared to pre-surgical baseline levels. In the majority of trials (7/9), microfracture was used as the control group. Although clinical outcomes varied between studies, microfracture was found to be either equivalent or inferior to OAT, ACI and MACI, but never superior. No significant difference was found between the failure rates of various techniques in any trial up to 5 years. However, as expected, failure rates increased with time, and a significant difference could be detected between treatment methods after 10 years, emphasising the importance of long-term follow-up (Table 5.3). Lesion size was determined to be important with the overall results of the systematic review suggesting improved short-term results with cartilage regeneration techniques (OAT, ACI and MACI) over MF in larger lesions (>4.5 cm2)[23, 79, 81, 84].
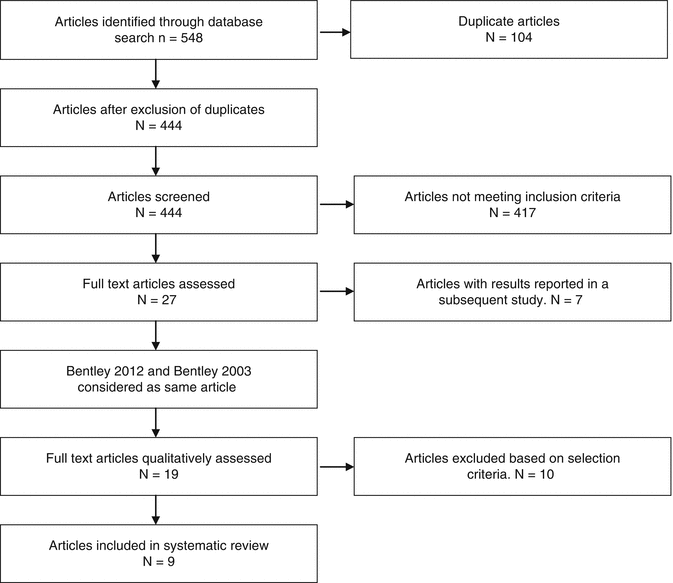
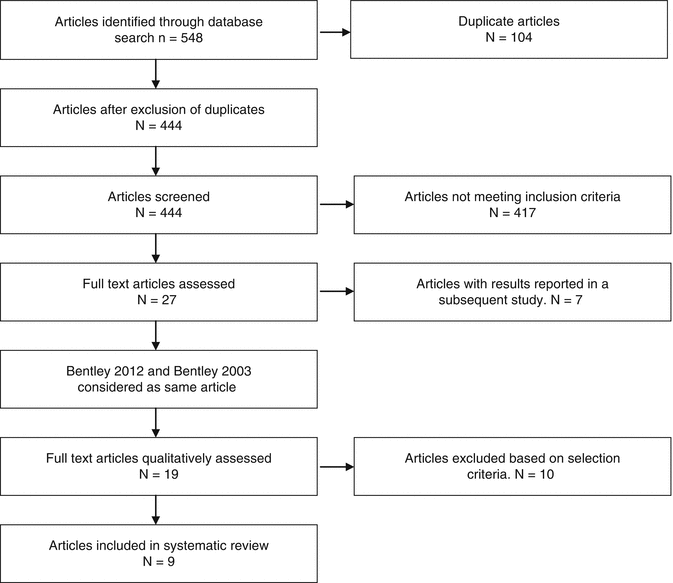
Fig. 5.5
PRISMA flowchart for systematic review of level I studies
Table 5.2
Cartilage technique failure rate in level I studies
Follow-up | Treatment | Failure | Significant difference | |
---|---|---|---|---|
Bartlett et al. [81] | 1 year | ACI | 0 | NS |
MACI | 2 of 47 (4 %) | |||
Bentley et al. [79] | 10 years | ACI OAT | 10 of 58 (17 %) 23 of 42 (55 %) | P < 0.001 |
Gudas et al. [82] | 10 years | OAT Microfracture | 4 (14 %) 11 (38 %) | P < 0.05 |
Gudas et al. [83] | 3 years | – | Not stated | – |
Knutsen et al. [23] | 5 years | ACI Microfracture | 9 (mean 26.2 months) 9 (mean 37.8 months) | NS (P = 0.101) |
Saris et al. [84] | 2 years | MACI Microfracture | 0 2 | NS |
Stanish et al. [30] | 1 year | – | Not stated | – |
Ulstein et al. [85] | 9.8 years | Microfracture
![]() Stay updated, free articles. Join our Telegram channel![]() Full access? Get Clinical Tree![]() ![]() ![]() |