© Springer Japan 2015
Kazuyuki Kanosue, Tomoyuki Nagami and Jun Tsuchiya (eds.)Sports Performance10.1007/978-4-431-55315-1_22. Brain Activity During Motor Imagery
(1)
Faculty of Sport Sciences, Waseda University, Saitama, Japan
Abstract
Motor imagery practice is useful for the acquisition of motor skills. Understanding the neural mechanisms underlying motor imagery is important not only for effective motor imagery practice but also for understanding the basic mechanisms involved with motor control. It is well documented that brain activity during motor imagery is similar to that which occurs during normal motor execution. This similarity supports the finding that motor skills can be acquired via motor imagery training. In this chapter, I will summarize available information on the brain activity that occurs during motor imagery.
Keywords
Motor imageryMental practiceBrain imagingFunctional magnetic resonance imagingCorticospinal excitability2.1 Introduction
Motor imagery is defined as the mental execution of a movement without any overt movement or muscle activation (Lotze and Halsband 2006). Motor imagery has been typically separated into two types “first person perspective imagery” and “third person perspective imagery”. Brain activities are different for these two types of imagery (Decety and Lindgren 1991; Munzert et al. 2008; Holmes and Calmels 2008). Motor imagery practice is useful for athletes, patients and healthy people in the acquisition of not only motor skills, but also muscle strength (Feltz and Landers 1983; Yue and Cole 1992; Driskell et al. 1994; Murphy 1994; Pascual-Leone et al. 1995; Stevens and Stoykov 2003; Mulder et al. 2004; Allami et al. 2008; Holmes and Calmels 2008; Gentili et al. 2010; Guillot et al. 2010; Mizuguchi et al. 2012a). These positive effects would be related to the fact that neural substrate during motor imagery sheres motor execution.
2.2 Measurement of Brain Activity
The motor imagery is based on brain activity in many regions and along numerous pathways. Techniques utilized to study imagery include functional magnetic resonance imaging (fMRI), positron emission tomography (PET), transcranial magnetic stimulation (TMS), magnetoencephalography (MEG), and electroencephalography (EEG).
2.2.1 fMRI and PET Studies
Numerous studies on imagery have utilized fMRI and PET. As previously mentioned, brain activity during motor imagery largely overlaps that which occurs during normal motor execution (Hanakawa et al. 2003; Lacourse et al. 2005; Lotze and Halsband 2006; Imazu et al. 2007; Hanakawa et al. 2008; Guillot et al. 2009;,Mizuguchi et al. 2013a). Areas activated during motor imagery include the supplementary motor area (SMA), the premotor cortex (PM), the primary motor cortex (M1), the parietal cortex, basal ganglia and the cerebellum (Decety et al. 1994; Lotze et al. 1999; Naito et al. 2002; Stippich et al. 2002; Hanakawa et al. 2003, 2008; Kuhtz-Buschbeck et al. 2003; Lacourse et al. 2005; Michelon et al. 2006; Lotze and Halsband 2006; Szameitat et al. 2007a, b; Higuchi et al. 2007; Imazu et al. 2007; Munzert et al. 2008; Chen et al. 2009; Guillot et al. 2009; Lorey et al. 2010; Mizuguchi et al. 2013a). The most consistent findings involved activation of the SMA, PM, parietal cortex and cerebellum. These regions certainly play major roles not only in normal motor execution but also in motor imagery. However, altered neural activity in many brain regions such as the precentral gyrus and cerebellun is lower during motor imagery than during the activation of actual movements (Hanakawa et al. 2003; Michelon et al. 2006). On the other hand, the superior parietal cortex is activated more during motor imagery than motor execution (Hanakawa et al. 2003). Motor imagery has also been implicated in the activation of other brain region, such as the dorsolateral prefrontal cortex (DLPFC), the putamen and the insula (Hanakawa et al. 2003; Szameitat et al. 2007a, b; Munzert et al. 2008; Guillot et al. 2009). However, the extent of activation of these areas differs across studies. These differences might be related to type of tasks imaged and subjects utilized.
2.2.2 TMS Studies
TMS has proven to be a valuable technique. TMS is performed by passing a high-current pulse through a magnetic coil placed on the scalp. Motor evoked potentials (MEPs) can be recorded from muscles activated by a single suprathreshold TMS pulse delivered to the contralateral primary motor cortex. The magnitude of the MEPs depends on the level of excitability of the corticospinal tract (Rothwell 1991; Hallett 2000; Reis et al. 2008).
Corticospinal excitability during motor imagery leads to increases above the normal resting excitability level. Kasai and colleagues (1997) demonstrated that the MEPs amplitude of the flexor carpi radialis muscle dramatically increased during imagery of wrist flexion. Subsequent studies have confirmed the phenomenon (Yahagi and Kasai 1998; Hashimoto and Rothwell 1999; Fadiga et al. 1999; Li et al. 2004a). However, the increase in excitability is smaller than that which occurs during actual movement activation (Li 2007). Corticospinal excitability during motor imagery of a movement follows the same temporal changes in muscle activities that occur during the actual execution of the movement (Hashimoto and Rothwell 1999). In addition, this enhancement of corticospinal excitability is related to the imagined force level (Mizuguchi et al. 2013b). This is another example of how, during motor imagery, the brain shows an activity pattern very similar to that which occurs during the elicitation of an actual execution. Abbruzzese and colleagues (1999) as well as Liepert and Neveling (2009) observed that during motor imagery, intracortical inhibition was decreased while intracortical facilitation remained unchanged. This finding suggests that the enhancement of corticospinal excitability that occurrs during motor imagery is caused by disinhibition (Takemi et al. 2013).
The difference in corticospinal excitability across individuals corresponds closely to differences in the vividness of their motor imagery as assessed by questionnaire. That is, subjects who can imagine a movement more vividly would also show an enhanced corticospinal excitability during motor imagery as compared to subjects with low imagery ability (Fourkas et al. 2008; Lebon et al. 2012; Williams et al. 2012).
2.2.3 MEG and EEG Studies
It is well known that internal and external events result in a change in many frequency bands of ongoing EEG signals. Depending on the nature of the alteration of the waveform, this is referred to as either event-related desynchronization (ERD) or event-related synchronization (ERS) (see review by Pfurtscheller and Lopes da Silva 1999). During motor imagery (kinesthetic imagery) of hand movement, ERD of 8–13 Hz (alpha bands) and 14–30 Hz (beta bands) in the contralateral hemisphere was observed (Nam et al. 2011). The ERD recorded from the MI associated with downregulation of intracortical inhibition during motor imagery (Matsumoto et al. 2010; Takemi et al. 2013).
Event-related potentials (ERP) have also been used in the investigation of motor imagery (Naito and Matsumura 1994; Romero et al. 2000). ERP, which are generally averaged EEG waveforms with respect to each event, reflect time-locked changes in brain activity (Picton et al. 2000). Naito and Matsumura (1994) measured a negative potential recorded at FCz in response to a visual cue with motor execution, motor imagery or No-go response. They demonstrated that the amplitude of the negative component was smaller in the imagery condition than in the execution condition. By contrast, the amplitude in the No-go condition was larger than that in the Go condition. Naito and Matsumuras’ findings suggest that neural processes of involved with motor imagery differ from simple motor suppression.
2.3 Role of Each Region
2.3.1 Supplementary Motor Area and Premotor Cortex
Most studies indicate that the SMA and PM are activated during motor imagery (Decety et al. 1994; Lotze et al. 1999; Naito et al. 2002; Stippich et al. 2002; Hanakawa et al. 2003, 2008; Kuhtz-Buschbeck et al. 2003; Lacourse et al. 2005; Michelon et al. 2006; Lotze and Halsband 2006; Szameitat et al. 2007a, b; Higuchi et al. 2007; Imazu et al. 2007; Munzert et al. 2008; Chen et al. 2009; Guillot et al. 2009; Lorey et al. 2010; Mizuguchi et al. 2013a). Taken together, these findings indicate the SMA and the PM are very likely to be essential parts of the neuronal network involved with motor imagery. Indeed, activity in the SMA and PM during imagery of the fingers, toes and tongue were activated in the area corresponding to the each body part (Ehrsson et al. 2003). The same corresponding areas were then activated during the actual execution. In addition, activity in the PM correlated with the vividness of motor imagery as assessed by a questionnaire utilizing a 7-point scale (Lorey et al. 2011).
By contrast, Kasess and colleagues (2008) investigated the effective connectivity during motor imagery utilizing fMRI and dynamic causal modeling analysis. Their results suggest that the activity in the SMA suppresses the activity in the M1. This conclusion indicates that the lack of activation in M1 during motor imagery could be caused by suppression emanating from the SMA (Kasess et al. 2008). A recent MEG study also reported that the activity in the SMA would inhibit the activity in the M1 during motor imagery (Di Rienzo et al. 2014). Therefore, the SMA apparently functions not only to generate motor representation but acts suppress the M1 during motor imagery in order to inhibit muscle contraction.
2.3.2 Parietal Cortex
Sirigu and colleagues (1996) evaluated the contribution of the parietal cortex to motor imagery by using mental chronometry in patients. They report that a patient with lesions restricted to this area of the cortex was not able to imagine an action accurately. According to Desmurget and colleagues (2009), stimulation of the inferior parietal lobule produced intention to move or patients reported to have moved, even in the absence of actual motor responses. Single-pulse TMS over the parietal cortex (superior parietal cortex), which can temporarily deactivate neurons in healthy subjects (virtual lesion) (Pascual-Leone et al. 2000), decreased the accuracy of motor imagery (Fleming et al. 2010). In this experiment, to assess the accuracy of motor imagery, subjects were asked to imagine a sequence of hand and arm movements given by verbal instruction, and then asked to tell the investigator the final position of the hand and arm. Subjects answered correctly if they were able to imagine the movements as the instructions progressed. These results would indicate that the parietal cortex plays an important role for the recognition of imagined action.
Recently, cortico-cortical connectivity between parietal and bilateral primary motor cortices was investigated during motor imagery by using a combination of TMS and transcranial direct current stimulation (tDCS) (Feurra et al. 2011). During motor imagery, anodal tDCS over the parietal cortex increased MEPs amplitude, but only from the ipsilateral M1 and not from the contralateral M1. These results suggest that a major role in motor imagery is restricted to the ipsilateral parieto-motor circuitry.
2.3.3 Primary Motor Cortex
While some researchers found M1 activation during motor imagery (Porro et al. 1996; Lotze et al. 1999; Chen et al. 2009; Guillot et al. 2009), others did not (Decety et al. 1994; Naito et al. 2002; Kuhtz-Buschbeck et al. 2003; Higuchi et al. 2007; Szameitat et al. 2007a). This discrepancy might be associated with such factors as the degree of muscle activity, type of tasks, and subjects (Munzert et al. 2009). Kuhtz-Buschbeck and colleagues (2003) investigated brain activity and corticospinal excitability using fMRI and TMS during motor imagery. They found a significant enhancement of corticospinal excitability with TMS, but not significant activation in M1 when utilizing fMRI. These findings indicated a possibility that sensitivity for the detection of neural activation, especially in the M1, was higher for TMS than fMRI.
The effect of physical practice on motor performance is higher 30 min after practice than right after practice (Debarnot et al. 2011). That is, skill acquisition occurs not only during physical practice but continue after the practice is over. This phenomenon is called “early consolidation” (Muellbacher et al. 2002). Early consolidation was also observed 30 min after a motor imagery practice (Debarnot et al. 2011). Inhibition of neuronal activity in M1 by a continuous theta-burst stimulation (cTBS) immediately after motor imagery practice blocked the early consolidation. These findings suggest that neuronal activity in M1 is involved in the motor learning that accrues to imagery practice.
2.3.4 Spinal Cord
It has been reported that motor imagery did not modulate spinal excitability as assessed by the H reflex (Abbruzzese et al. 1996; Aoyama and Kaneko 2011) or F wave (Facchini et al. 2002). By contrast, the stretch reflex was augmented during motor imagery (Li et al. 2004b; Aoyama and Kaneko 2011). Aoyama and Kaneko (2011) clarified the effect on this potential by demonstrating that the amplitude of the stretch reflex was increased during motor imagery of dorsiflexion and plantarflexion, but the amplitude of H-reflex was not altered. Thus, while motor imagery seems to increase the excitability of some spinal reflexes, others are unaffected. At present, the nature of spinal cord involvement with motor imagery is unclear.
2.4 Influence of Somatosensory and Visual Information on Motor Imagery
Somatosensory input interacts with motor imagery (Naito et al. 2002; Thyrion and Roll 2009). Naito and colleagues (2002) report that the kinesthetic illusion elicited by tendon vibration (proprioceptive input) of the wrist extensor is enhanced by motor imagery of wrist flexion. The actual hand posture also affects brain activity during motor imagery (Vargas et al. 2004; Fourkas et al. 2006; Linag et al. 2007). For example, Vargas and colleagues (2004) showed that corticospinal excitability during imagery of finger-thumb opposition was larger when the actual posture was the same as the imagined (congruent) hand than when it was different from the imagined (incongruent) hand. This result suggests that proprioceptive inputs which are congruent with imagined actions enhance corticospinal excitability during motor imagery. However, the effect of posture on motor imagery seems to depend on the type of motor imagery (kinesthetic or visual), because posture has been found to affect corticospinal excitability during kinesthetic imagery, but not during visual imagery (Fourkas et al. 2006).
In many sports various tools and objects such as balls, gloves, bats and rackets are used. In the process of manipulating the objects, tactile information provides an important input. In such motor imagery, tactile input generated by passively touching an object was found to increase corticospinal excitability (Mizuguchi et al. 2009, 2011, 2012b). This enhancement of corticospinal excitability was not merely the effect of just holding something, in this case a ball, because corticospinal excitability did not increase while holding the object without motor imagery. Further, when the actual posture was different from the imagined action, tactile input did not increase corticospinal excitability (Mizuguchi et al. 2011). These results suggest that corticospinal excitability during imagery with an object is affected by the actual touching of the object and is thus modulated via combination of tactile and proprioceptive inputs.
Visual information also influences corticospinal excitability during motor imagery. Sakamoto and colleagues (2009) demonstrated that corticospinal excitability during simultaneous observation and imagery of elbow flexion was facilitated as compared to the excitability that occurred during observation or imagery alone. However, facilitation due to the combination of observation and imagery was not obtained when the participants imagined an action that was out of phase with the actual observation. Therefore, visual input that is congruent with an imagined action is likely to increase corticospinal excitability during motor imagery. This evidence suggests that somatosensory and visual inputs which temporally closely resemble the actual execution increase the effectiveness of motor imagery. This implies that appropriate input selection is critical in maximizing the effectiveness of motor imagery in training or rehabilitation.
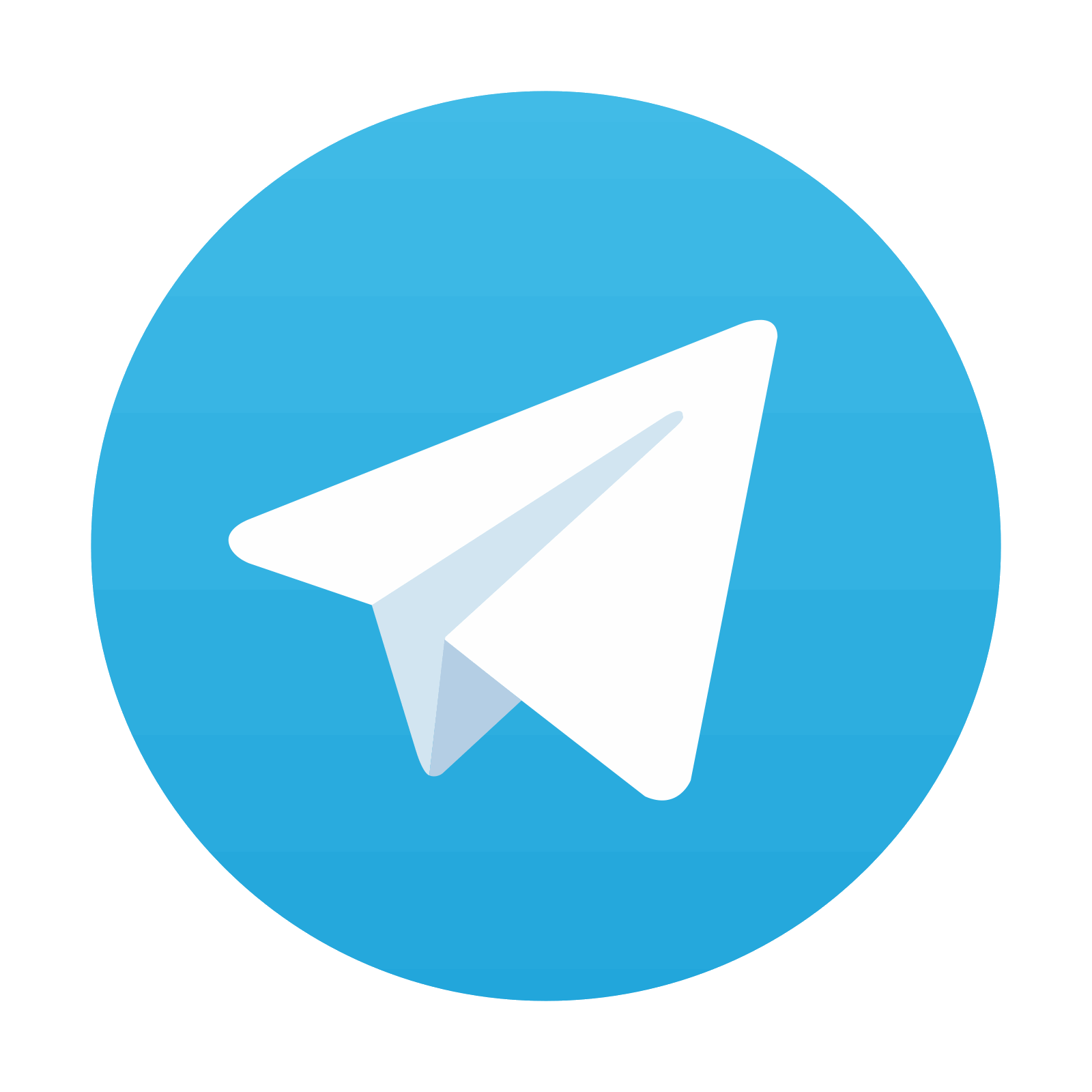
Stay updated, free articles. Join our Telegram channel
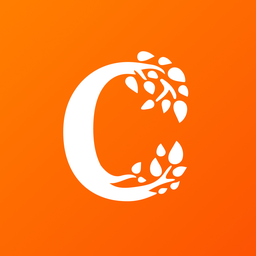
Full access? Get Clinical Tree
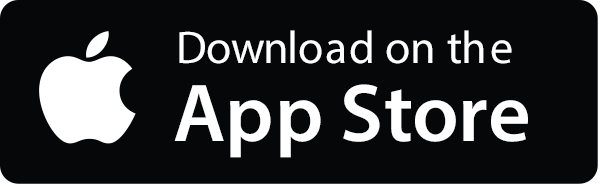
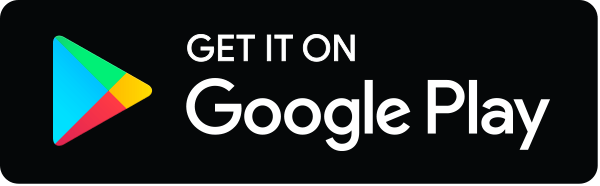