Bone Remodeling Around Hip Implants
Ryan D. Ross
Margaret A. McNulty
Rick R. Sumner
Introduction
The term “bone remodeling” refers to the coupled process of bone resorption and bone formation, in which the amount of extracellular matrix removed by osteoclasts in a particular anatomical location is normally balanced by the amount of matrix subsequently deposited by osteoblasts (1,2). In orthopedics, the term is often used to describe the outcome from the process (e.g., loss of peri-implant bone mass), but here we will distinguish the process from the result to help clarify what is known about underlying mechanisms. Bone remodeling is of interest because aseptic loosening, which is a major complication in total joint replacement (TJR), occurs when there is early failure to achieve or later failure to maintain implant fixation. These failures are thought to be direct or indirect consequences of peri-implant bone remodeling.
Three phenomenon affected by bone remodeling have raised the most attention in the context of total hip arthroplasty (THA); osseointegration, osteolysis, and stress shielding (Fig. 48.1). Osseointegration refers to direct attachment of the implant to the host skeleton, which is important for stabilizing the implant mechanically (3). Long-term maintenance of an osseointegrated implant depends upon maintenance of viable bone at the interface. Osteolysis is an example of pathologic remodeling because it occurs as a response to wear or other particulate debris shed from the implant. In the case of osteolysis, bone resorption outpaces bone formation and considerable bone mass is lost (4), placing the patient at increased risk of peri-implant bone fracture or implant loosening and often necessitating revision surgery. Stress shielding, which refers to a reduction in mechanical stimulus to the surrounding bone because of the presence of the implant (5,6,7), is thought to cause adaptive remodeling, a nonpathologic response to the change in the bone’s mechanical environment. Stress shielding leads to bone loss in some locations about the implant and bone formation in others, although the relative contributions of changes in bone resorption and formation are not well defined (8).
The current annual estimated costs of aseptic loosening in the United States may be as high as $10B and affects thousands of patients (9,10,11). Current clinical management is largely based on surgical intervention. The number of TJR revision surgeries performed annually in the United States is well over 70,000 and the annual incidence is expected to increase to more than 350,000 by 2030 (12), with aseptic loosening being one of the major indications for revision. These statistics are particularly worrisome because of the relatively high failure rates of revision THA, ranging from 10% to 25% (13,14).
As the age of individuals receiving THA declines and the life expectancy of these patients continues to rise, it is important to understand how and why hip implants fail. The bone remodeling process has been studied extensively in the context of metabolic bone diseases such as osteoporosis, but much less attention has been paid to details of remodeling in the context of joint replacement. Within this chapter we will concentrate on bone remodeling related to osteolysis and stress shielding with a brief review of osseointegration. It is our hope that an improved understanding of peri-implant bone remodeling will lead to improved initial fixation and prevent later loss of fixation, thereby reducing the need for revision surgeries.
What Causes Peri-Implant Bone Remodeling?
Osseointegration and the Initial Response to Surgery
Osseointegration is defined as the direct contact of bone with the surface of the implant material (3). This bone–implant contact helps to stabilize the implant within the host bone and can prevent micromotion, or microscale movement of the implant relative to the host bone, which is thought to play an important role in the eventual failure of joint arthroplasties (15). Using design parameters and biologic treatments, scientists have been able to increase the bone formation which stabilizes implants (16,17). In the clinical setting, the most commonly used strategies to enhance osseointegration are to use an implant that is inherently stable mechanically with good initial contact between the implant and host bone and to use surface treatments favorable to extensive bone–implant ingrowth (16).
Bone remodeling adjacent to the implant begins almost immediately after surgery and overlaps chronologically with the injury response. In fact, the initial injury response is critical to establishing osseointegration (16). Thus, especially in cementless implants, the ability to obtain a strong mechanical bond between the implant and host bone depends upon the formation of woven bone at the interface, which is subsequently replaced by lamellar bone. Although cemented implants have their peak integration strength at the time of implant placement, it takes several weeks for implant fixation strength to fully develop in cementless implants and this process depends upon the ability of the body to form new bone and to form new bone and grow into or onto the implant surface (16).
The surgical procedure itself, beyond creating a stimulus for bone repair at the interface, also affects the surrounding host bone (18,19,20). A recent study showed that techniques used in femoral canal preparation affect the degree of porosity developed in the cortical bone, leading to an early loss in cortical bone mass (20). For instance, reaming increased porosity in a canine model by six-fold 6 weeks after surgery. The remodeling stimulus was presumably related to an interruption of the intramedullary blood supply, although heat generation may also contribute. A recent study from our laboratory showed that elevations in peri-implant trabecular and endocortical bone remodeling were transient in an unloaded rat intramedullary implant model (21). Thus, it is clear that canal preparation and implant placement trigger a remodeling response. It is not yet clear if these changes have downstream effects by directly or indirectly influencing later events such as osteolysis or stress shielding. It is possible that these remodeling transients may affect longitudinal changes in peri-implant bone mass and partially explain reported periods of initial bone loss, followed by recovery, often times followed in turn by longer-term bone loss (e.g., see (22,23)).
Stress Shielding and Adaptive Bone Remodeling
Stress shielding refers to the reduction in mechanical stimulus to peri-implant bone because the implant transfers the joint reaction force rather than the host bone (Fig. 48.2). Femoral hip stems are loaded in bending and therefore the bending stiffness of the implant relative to the bone is a key factor (24). Bending stiffness depends upon the modulus of elasticity of the material (E) and the area moment of inertia (I) of the structure. Femoral stems are made from materials that have an elastic modulus that is roughly five times (titanium and its alloys) or 10 times (cobalt-chrome) greater than the elastic modulus of cortical bone. Even though the bone may have a larger moment of inertia, the bending stiffness (E × I) of the implant is large enough, relative to the bone, to lead to a significant part of the load being transmitted by the implant (25,26). According to Wolff’s Law, bone adapts its shape to best accommodate the mechanical stresses experienced by the tissue (27). Thus, changes in the bone’s mechanical environment because of stress shielding have been correlated with the pattern of bone loss and bone gain following THA, much like the reduction in mechanical stress caused by weightlessness in astronauts causes bone loss (28). In the context of THA, bone
loss is particularly noticeable in regions that experience the greatest reduction in mechanical stimulation such as the calcar region of the proximal femur. Various design parameters such as the relative bending stiffness of the implant compared to the host bone are determinants of the severity of stress shielding and the amount of subsequent bone loss. Although stress shielding is thought to primarily affect cementless implant designs, cemented implants can also be affected (29). Most attention has been paid to loss of bone mass, but there is evidence that bone shape (30) and bone quality (31) can also be affected. In addition to stress shielding, other factors can contribute to the altered mechanical environment in the peri-implant tissue following THA, including altered loading caused by changes in gait (32), subject activity level (33), and the use of muscle splitting as opposed to muscle-sparing surgical approaches (34).
loss is particularly noticeable in regions that experience the greatest reduction in mechanical stimulation such as the calcar region of the proximal femur. Various design parameters such as the relative bending stiffness of the implant compared to the host bone are determinants of the severity of stress shielding and the amount of subsequent bone loss. Although stress shielding is thought to primarily affect cementless implant designs, cemented implants can also be affected (29). Most attention has been paid to loss of bone mass, but there is evidence that bone shape (30) and bone quality (31) can also be affected. In addition to stress shielding, other factors can contribute to the altered mechanical environment in the peri-implant tissue following THA, including altered loading caused by changes in gait (32), subject activity level (33), and the use of muscle splitting as opposed to muscle-sparing surgical approaches (34).
Particle-Induced Osteolysis
Particle-induced osteolysis is widely accepted as a key factor in aseptic loosening (35,36,37), although lack of initial mechanical stability and other factors may also play a role (15,38,39). Wear debris as well as other particles and ions released from implants are thought to invoke an immune system response through toll-like receptors 2 and 4 and the NALP3 inflammasome (40,41,42). Endotoxins (such as lipopolysaccharide) can potentiate the ability of particles to induce an inflammatory response (43). The potential role of adaptive immunity is not clear (44,45). Collectively, the inflammatory responses increase osteoclastogenesis and bone resorption and may also induce osteoblast apoptosis (42). In addition to these inflammatory pathways, particles can directly downregulate collagen type I production by osteoblasts (46), negatively affect osteogenic lineage cell proliferation and differentiation (47), and can induce a catabolic phenotype in osteoblasts and osteocytes (48,49). More detailed consideration of the cascade of events that occur during particle-induced osteolysis is beyond the scope of this chapter, but can be found in several review papers (42,50,51,52) as well as in Chapter 21 in this book.
Osteolysis, stress shielding, and osseointegration are all associated with changes in the bone remodeling process that occurs following THA and probably do not act independently (Fig. 48.1). Stress shielding, for instance, has been theorized to facilitate particle-induced osteolysis (53,54,55), by opening the bone–implant interface, thereby allowing for migration of particles within the intramedullarly canal to trigger osteolysis and peri-implant remodeling (56,57). Therefore, as the formation of a strong bone–implant interface can limit the migration of particles along the implant surface, maintenance of the interface through osseointegration is important to prevent failure of implant fixation (58,59,60,61). Perhaps, equally important is maintenance of peri-implant bone mass by reducing stress shielding and the accompanying loss of bone.
Clinical Detection and Evaluation of Peri-Implant Bone Remodeling
In this section, we summarize the primary methods used clinically to evaluate peri-implant bone remodeling. In almost all cases, the consequence of remodeling (e.g., change in bone mass) and not the remodeling process itself is characterized. There are some exceptions where attempts are being made to more directly assess bone remodeling, for instance, by using positron emission tomography (PET) for measuring bone formation activity locally and by examining biomarkers (see Section “Clinical detection and evaluation of peri-implant bone remodeling”).
Radiography
Radiography is the mostly commonly used method of evaluating the skeleton noninvasively and the technique has been commonly used to monitor changes in the bone following THA (62,63). Radiographs are simple to perform, inexpensive and relatively safe to the patient, and therefore are routinely used today. Radiographic evaluation is primarily qualitative and large amounts of bone must be lost before the presence of depressed bone mass can be inferred, therefore making a consensus definition of implant failure difficult (64,65). Radiographic evaluation of THA is a two-dimensional technique in which regions of lost bone are identified by the presence of relatively radiolucent areas, which in the extreme can indicate the presence of osteolytic lesions, that is, areas largely devoid of bone surrounding the implant (Fig. 48.3).
Dual-Energy X-Ray Absorptiometry
Dual-energy X-ray absorptiometry (DEXA) is used to investigate bone mineral density (BMD) in patients noninvasively. BMD is actually the bone mineral content (BMC) divided by the projected area of interest. Thus, this is not a true density measurement as the units are g/cm2. DEXA scans are the gold standard for the diagnosis of osteoporosis and low BMD scores have been shown to be positively correlated with an increased risk of fracture (66,67). The use of DEXA to measure periprosthetic BMD has been validated as an accurate and reproducible technique (68,69). Seven periprosthetic “Gruen zones” were first defined in a radiographic study of the bone–implant interface to provide anatomic context (62) and these zones have become the standard for reporting DEXA results (Fig. 48.4). Following THA, BMD is not lost homogeneously, and therefore, the Gruen zones are useful to provide an anatomically based coordinate reference system for the femur. The Gruen zones most commonly associated with stress shielding are the proximal most zones, particularly zone 7, or the calcar region and zone 1, which encompasses the greater trochanter. These regions have been shown to be the primary sites of net bone loss following implant placement and simulation studies have validated the mechanical unloading, or stress shielding, of these regions, as detailed below. In THA patients, decreased BMD can be caused by both stress shielding and osteolysis, but nearly all DEXA studies list the presence of osteolytic lesions as an exclusion criterion, so DEXA is almost always used to examine adaptive remodeling.
Prospective DEXA studies in which periprosthetic bone loss was directly inferred by comparing early postoperative scans to subsequent scans have shown that most of the bone loss occurs within the first 12 months, followed by a leveling off for 5 to 6 years, with evidence of continued bone loss in
subsequent years in Gruen zone 7 (Fig. 48.5) (22,70,71,72,73,74,75,76,77,78,79,80,81,82,83,84,85,86,87,88,89,90,91,92,93,94,95,96). Although the early impression was that measurable bone loss only occurred within the first 12 months (76,84,97) and was stable after 1 year (87,92,98), it is now clear that longer-term periprosthetic bone loss does occur. For instance, a 14-year longitudinal study (95) showed that BMD in Gruen zone 7 appeared to be stable from 6 through 24 months and then linearly decreased over the next 150 months. Another long-term study has shown rapid bone loss in the first year, with relative stability through 6 years, followed by continued bone loss up to 10 years (93). Between 12 and 17 years following cementless THA, a recent study has shown slight loss in Gruen zone 7 in men and losses in Gruen zones 1, 4, 6, and 7 in women (99). In some cases, BMD has been reported to increase after an early decline (75). Studies of bone changes adjacent to the acetabular component by DEXA are relatively rare along with reports of considerable bone loss (89) and small or no changes (100).
subsequent years in Gruen zone 7 (Fig. 48.5) (22,70,71,72,73,74,75,76,77,78,79,80,81,82,83,84,85,86,87,88,89,90,91,92,93,94,95,96). Although the early impression was that measurable bone loss only occurred within the first 12 months (76,84,97) and was stable after 1 year (87,92,98), it is now clear that longer-term periprosthetic bone loss does occur. For instance, a 14-year longitudinal study (95) showed that BMD in Gruen zone 7 appeared to be stable from 6 through 24 months and then linearly decreased over the next 150 months. Another long-term study has shown rapid bone loss in the first year, with relative stability through 6 years, followed by continued bone loss up to 10 years (93). Between 12 and 17 years following cementless THA, a recent study has shown slight loss in Gruen zone 7 in men and losses in Gruen zones 1, 4, 6, and 7 in women (99). In some cases, BMD has been reported to increase after an early decline (75). Studies of bone changes adjacent to the acetabular component by DEXA are relatively rare along with reports of considerable bone loss (89) and small or no changes (100).
DEXA measurements have limitations, as the technique is highly dependent on the placement of the patients within the scanner. Slight changes in the hip angle can have a dramatic effect on the resulting BMD outcome (68). In addition, BMD can be highly variable between individuals within a population, and therefore it has become a standard practice to use early postoperative BMD as the baseline for accurate prospective determination of implant effects (92). Because BMD is the ratio of BMC to the projected bone area, in cases where BMD has changed it is always wise to examine the BMC and area measurements, as well, particularly because the area measurement tends to change in Gruen zone 7 through “rounding off” of the calcar. Unfortunately, these data are rarely reported. Finally, having DEXA measurements from elsewhere in the body (e.g., spine or even the femur, well distal to the implant) are helpful in interpreting the BMD data from the bone adjacent to the implant and its relationship to age-related bone loss, but again, are rarely included in the literature.
![]() Figure 48.5. Change in Gruen zone 7 BMD as determined by DEXA as a function of length of follow-up. A: The data points represent the last follow-up in prospective studies involving either cemented or cementless implants (22,70,96). Note that most bone loss occurs early, followed by a period of stasis and subsequent further decrease. B: The data from seven of these studies are plotted so that the time course of change can be better appreciated (22,71,72,90,92,93,95). Three studies involved cemented implants and the remainder involved cementless titanium alloy or cobalt-chrome alloy implants. The two longest data points are inferred from long-term cross-sectional data associated with the prospective studies. These studies indicate that there can be late loss of peri-implant bone or even some gain in bone after an initial loss. |
Computed Tomography
Dynamic Bone Scanning
Bone turnover and perfusion have been examined through imaging of the bone-seeking radiotracers, 99mTc-labeled diphosphonates and 18F-NaF, for diagnosis of various skeletal pathologies (104) and may prove to be a valuable research tool for studying peri-implant bone remodeling. Recently, the combination of PET with CT has shown that remodeling activity can be anatomically localized through 18F-NaF imaging. For instance, studies using this imaging modality have suggested that bone metabolism returned to normal levels adjacent to cementless acetabular components 1 year after surgery (105), while activity adjacent to the femoral component tended to be higher at 4 months post surgery than at either the immediate postsurgical period or at 1 year (106). 18F-NaF PET imaging combined with DEXA has been used to infer that bone resorption led to continued loss of peri-implant bone mass even in the presence of elevated bone formation following revision THA (107). These methods have also been used to discriminate between aseptic and septic loosening (108).
Biochemical Markers
Bone remodeling is a cell-mediated process and monitoring the biochemical activity of the cells involved in this process presents an opportunity to assess the dynamic status of periprosthetic bone. The concentration of biochemical markers in body fluids has been shown to change as early as 3 to 6 months postoperatively, while radiographic evidence of bone loss can take up to 36 months to become apparent (109). The ability to diagnose excessive remodeling activity prior to the loss of considerable bone mass is particularly attractive as it may help to identify patients in need of pharmacologic intervention (see below) or allow for early surgical intervention. Therefore, the use of biochemical markers presents an opportunity for improved diagnostic evaluation of periprosthetic bone remodeling and subsequent implant loosening (110). The studies that have evaluated the concentration of biomarkers commonly do so by determining the utility of the marker of interest for the diagnosis of implant loosening and as such the biomarker concentrations are often correlated to radiographic evidence of osteolysis (Table 48.1).
Overall, biomarkers of bone formation, such as osteocalcin (OC), bone-specific alkaline phosphatase (b-ALP), and collagen propeptides, PINP and PICP, have had limited utility in predicting implant failure (Table 48.1). Although, the concentrations of these markers tend to change following THA, the direction, magnitude and significance of the changes are variable. Biomarkers of osteoclast-mediated bone resorption including NTX (74,111,112,113) and TRAP 5b (110,114,115), in particular, have successfully demonstrated correlation with clinical loosening.
Longitudinal studies of biomarkers have demonstrated the transient nature of the cellular activity following THA (109,118,119,120). In general, resorption markers tend to peak shortly after implant placement, and formation markers seem to initially decrease with increasing concentration developing thereafter. Therefore, it is important that the time of sampling be chosen to best monitor the biomarker of interest. Biomarker concentrations have also been shown to have high interindividual variations related to age, gender, disease, and menopausal status. Therefore, it is important to determine baseline marker concentrations prior to implant surgery (121,122,123). In addition, preoperative biomarker concentrations themselves may have predictive value for the determination of patients at risk to develop loosening (74,124).
Table 48.1 Biochemical Markers of Implant Loosening | ||||||||||||||||||||||||||||||||||||
---|---|---|---|---|---|---|---|---|---|---|---|---|---|---|---|---|---|---|---|---|---|---|---|---|---|---|---|---|---|---|---|---|---|---|---|---|
|
Evaluation of Disease Mechanisms
Measuring periprosthetic bone remodeling clinically is a challenge. Direct assessment is generally only possible in retrieval studies in which the bone and implant are removed and sectioned for histologic assessment (125). These studies are by definition retrospective, and if used as the sole method for assessment would make innovation time-prohibitive. Therefore, the understanding of many of the biologic mechanisms associated with bone remodeling, and methods to limit the remodeling process, have initially been assessed in the laboratory setting. In vitro cell studies, for instance, have been critical in the understanding of the biologic cascade triggered by wear particles (126,127,128). Animal models provide an opportunity to test treatment options, evaluate new designs or materials, or to determine the role of genes responsible for the remodeling response. Computer simulations, using techniques such as finite element (FE) analysis, allow for modeling of the loading patterns expected following THA and can be used to optimize implant designs. Some examples of the information obtained from these various studies are outlined below.
Retrieval Studies
Implants that were retrieved because of failure at the time of revision surgery (129
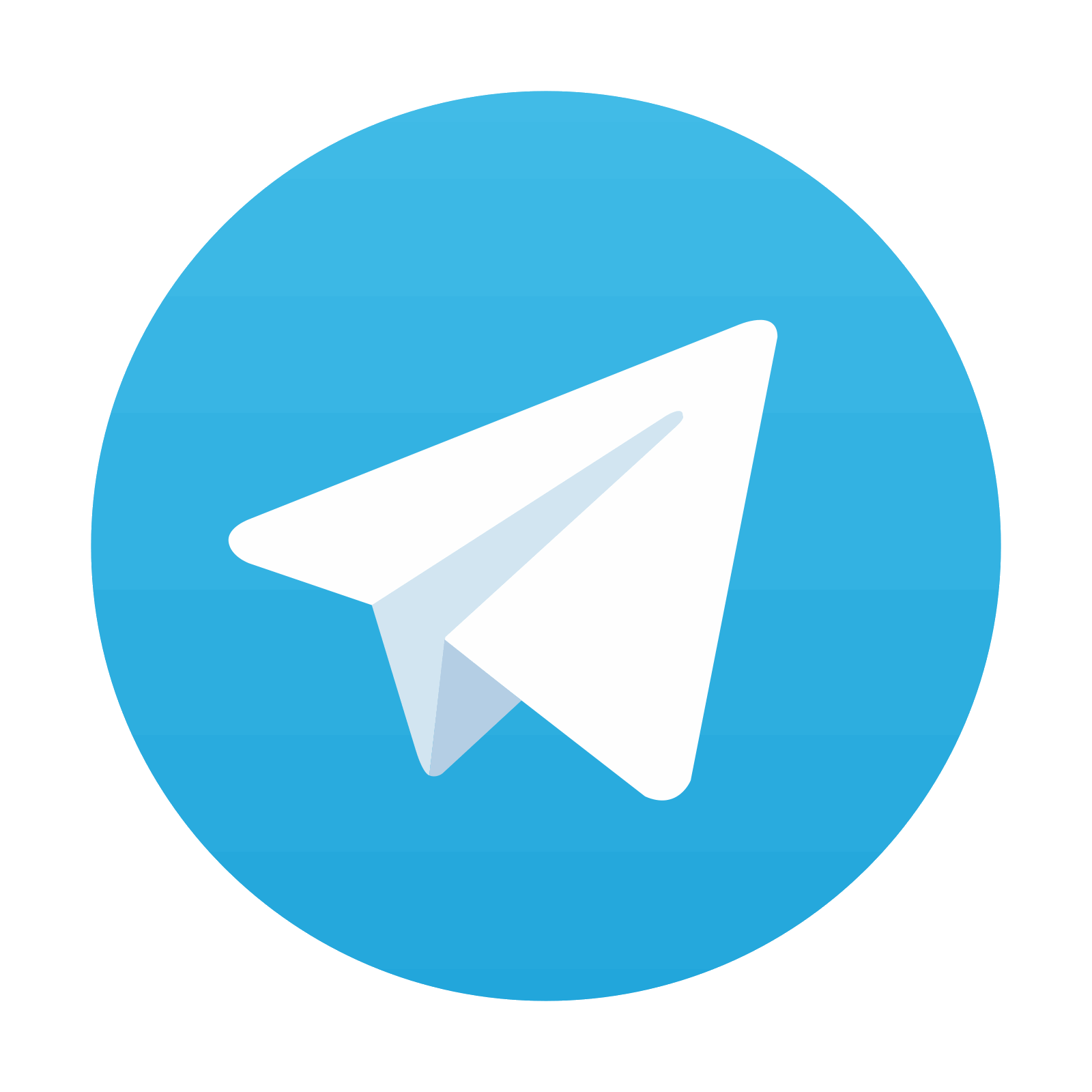
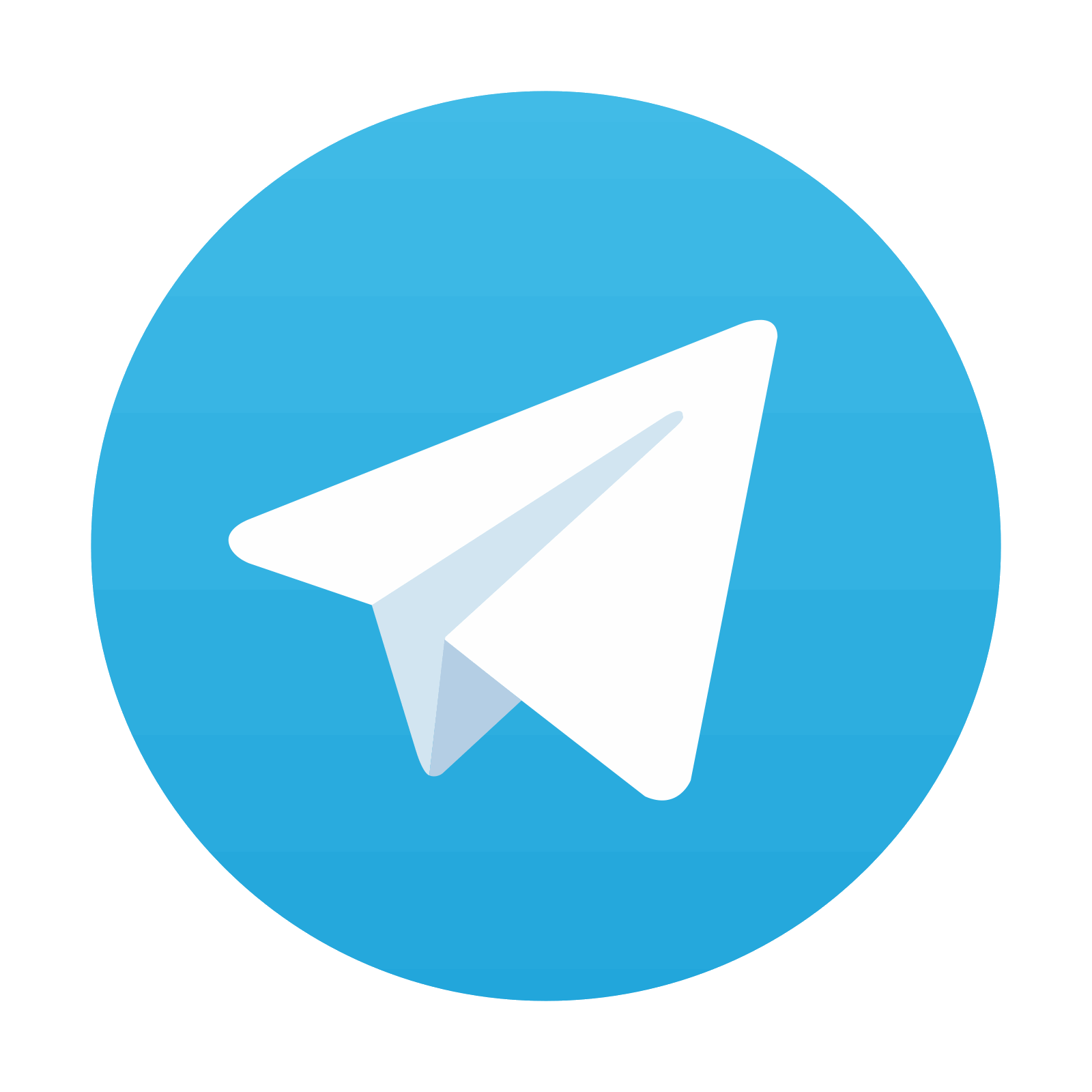
Stay updated, free articles. Join our Telegram channel
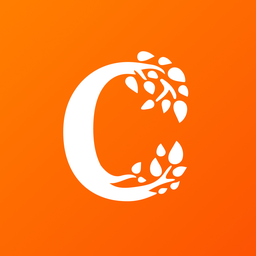
Full access? Get Clinical Tree
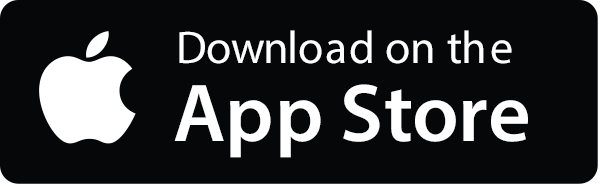
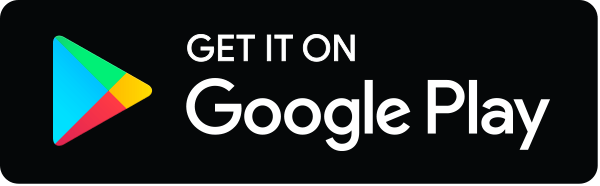
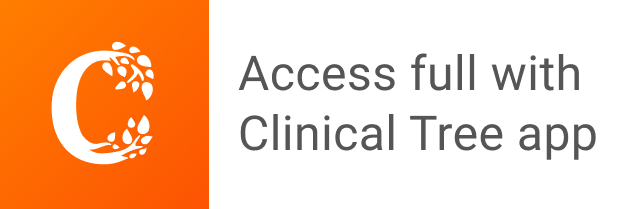