Fig. 9.1
Bioreactors diagram for bone tissue engineering. (a) Spinner flask; (b) Rotating wall bioreactor; (c) Flow perfusion bioreactor (From Olivier et al. [9], with permission)
Rotating Bioreactor
The rotating bioreactor (Fig. 9.1b) is developed to engineer cartilage and bone. There are three kinds of geometries: the slow-turning lateral vessel (STLV), the high-aspect-ratio vessel (HARV) and the rotating-wall perfumed vessel (RWPV). They are composed of two concentric cylinders. The cell construct could float in the annular space between the two cylinders. The microgravity environment is produced by the dynamic laminar flow, which is created by the rotating of the cylinder. The gas exchange is performed via internal or external membrane. Medium is exchanged batchwise or by periodic medium recirculation. The rotating bioreactor provides a hydrodynamic environment which is suitable for bone [11, 12] and cartilage tissue growth and differentiation [13]. However, the environment in the spinner flask bioreactor seems to favor the MSCs differentiation than in the RWV. Sikavitsas et al. [14] compared three different culturing conditions (spinner flask culture, static culture and rotating wall vessel culture) for their ability to promote the proliferation and differentiation of marrow stromal osteoblasts seeded on PLGA porous scaffolds. The results showed that the potential mitigation of external mass transport limitations in the spinner flask culture could have beneficial effects on the proliferation and differentiation of marrow stromal osteoblasts seeded on PLGA porous scaffolds.
Perfusion Bioreactor
The perfusion bioreactor is different from the above two bioreactors. It usually includes a pump, a reservoir, a chamber and the connecting tubes. The medium in the reservoir is pumped continuously into the chamber which contains the cell construct by a peristaltic pump [4]. Originally, the medium is passed around the edge of the scaffold and the bioreactor is called ‘perfusion chamber’. However, the medium in the center of the scaffold could not be exchanged sufficiently in the perfusion chamber. In 2003, Bancroft et al. [15] introduced a new bioreactor (Fig. 9.1c) in order to confine the medium to pass through the interconnected porous network in the scaffold. The new system guaranteed an exchange of medium within the porous scaffold. The perfusion bioreactor could avoid the inappropriate shear stress created by the mechanical mixing in the rotating bioreactor or spinner bioreactor. Further study demonstrated that flow perfusion augmented the functionality of scaffold/cell constructs grown in vitro as it combined both biological and mechanical factors to enhance cell differentiation and cell organization within the construct [16]. The environment (e.g. pH, temperature, pressure, nutrient supply and waste removal) in the bioreactor can be precisely controlled and monitored. However, most perfusion systems are designed for culture small cell construct, which could not fulfill the need of large scale bone defect [17].
In 2006, Xie et al. [18] designed a simple novel perfusion bioreactor (Fig. 9.2) for engineering large scale cell construct. The system is composed of three parts: a peristaltic pump, a 75-cm2 flask containing the perfusion medium and the tubes. One end of the silicone tubes penetrates through the plug cap into the flask as the inlet or outlet. Each cell construct is incubated separately and independently. The seeded scaffold is connected with the silicone inlet and is put into the flask and suspended in the perfusion medium which is over the top of scaffold. The perfusion bioreactor formed bionic circular system. The porous β-TCP scaffold has a central tunnel with blind end. The tunnel connects with the tubing system. The macropores open into this tunnel on the lateral wall. All the macropores are well interconnected mimicking the microcircular system. The macropores also open into the medium through the lateral pore on the outer surface of the scaffold. Then the medium could be continually drawn from the reservoir and pumped into the scaffold through the central tunnel. Thus, the metabolic waste in the macropores is brought away. The fresh nutrition and oxygen are brought in. The medium is changed batchwise. The cells inside the scaffolds could survive and proliferate. This kind of built-in flow path not only assures the medium to be perfused into the scaffold, but also makes it possible to utilize the scaffolds with different size and shape. The latter is one of the prerequisites for using custom-made large scale scaffold, especially for reconstruction after bone tumor resection.


Fig. 9.2
Perfusion bioreactor diagram for large scale cell construct (From Xie et al. [18], with permission)
Roles of the Bioreactor in Bone Tissue Engineering
Cell Seeding
Cell seeding is the first step before engineering bone tissue. In this stage, the MSCs have to be loaded in the porous scaffold homogeneously. It is supposed that the greater number of cells loaded in the scaffold will increase bone mineralization [19]. Moreover, the spatial distribution of cells within the scaffold after seeding has an effect on the final engineered tissue [20, 21]. Hence, effort has been made to optimize the seeding method in order to achieve the initial construct with uniform and highly dense cells.
Static loading is the traditional way to seed the cells into the scaffold. In this fashion, the single cell suspension solution is prepared before loading. The solution is dropped into the scaffold. Or the porous scaffold is put in the solution directly. The vacuum is often produced by a pump to draw the air out of the scaffold and to make the cell solution penetrate into the scaffold. However, the resulting cell construct is not homogeneously, especially when the scaffold is large enough. The cell density on the surface of the scaffold is much higher than that in the center of the scaffold. Previous study showed that with the static loading, the seeding efficiencies are low and the cell distribution is not homogenous in the scaffold [19, 21–24].
Dynamic seeding is to seed the cell into the scaffold with dynamic flow. It has been reported that dynamic seeding yielded higher seeding efficiency and homogeneity than the static seeding. With the spinner bioreactor, the high and spatially uniform distribution of chondrocytes was achieved in highly porous, fibrous polyglycolic acid scaffolds for rapid and uniform tissue regeneration [25]. Du et al. [26] and Wendt et al. [27] developed an oscillatory perfusion bioreactor. The oscillating perfusion of cell suspensions through three-dimensional scaffolds yielded the higher seeding efficiency, and homogeneous scaffold cellularity throughout the scaffolds compared with either static seeding or the stirred-flask bioreactor. Li et al. [23] found that the dynamic depth-filtration seeding method was better in providing a higher initial seeding density and more uniform cell distribution and was easier to apply to large tissue scaffolds. A depth-filtration model was developed and could be used to simulate the seeding process and to predict the maximum initial seeding densities in matrices with different porosities. Nowadays, the perfusion seeding system has become part of a perfusion bioreactor system. This facilitates the seeding procedure and the subsequent culture procedure. Thus it reduces the possibility of contamination during the seeding and culture procedure.
Mass Transfer
After the seeding, the cell constructs have to be cultured in vitro for a period before the formation of the bone tissue. During this stage, the cells will proliferate and differentiate. The sufficient supply of oxygen and nutrient in the scaffold is the fundamental issue for cell survival and proliferation. Previous study on the tumor cellular spheroids showed that the spheroid larger than 600 μm in diameter generally contained a severe hypoxic center and the cells could only survive in the periphery [28]. The cells only survive and proliferate in the exterior pores of the 3-D scaffold under the static culture. Under static culture condition, the mass transfer depends on the gradient difference of mass concentration. The diffusion rate of mass transportation in the static culture was only sufficient to make the cells survive about 500–1500 μm under the scaffold surface [6, 29, 30]. Therefore, the cells proliferated sufficiently only in the macropores near the scaffold surface. Because the size of the engineered constructs is often too large to be cultured in a traditional way. The improvement of mass transfer is one of the challenges in the progress of bone tissue engineering.
Dynamic culture created by the bioreactor gives an answer to this problem. In the spinner flask, the oxygen and nutrients in the medium are mixed continuously. The concentration boundary layer at the construct surface also changes and the mass transfer is improved. It is supposed that the better mixing provided in the spinner flask, external to the outer surface of the scaffolds, enhances the proliferation and differentiation of marrow stromal osteoblasts [14].
In the rotating bioreactor, a dynamic laminar flow is generated and the mass transfer improved. It has been proved that the chondrocytes construct cultured in rotating bioreactor is superior to that cultured in the spinner flask or cultured statically [31]. The flow velocity around and through the scaffolds in rotating bioreactors can be manipulated and the 3D dynamic flow environment affects bone cell distribution in 3D cultures and enhances osteoblastic cell phenotypic expression and mineralized matrix synthesis within tissue-engineered constructs [11].
In the perfusion bioreactor, the medium is not only pumped into the reservoir but also perfused through the interior path in the scaffold. The interior passage for the fluid is composed of the macropores and the interconnections in the scaffold. This interior passage is also used for the cell adhesion and proliferation. Under dynamic perfusion culture the gas exchange and nutrition supply are improved in the center of the scaffold as well as in the peripheral part. The waste can be brought away directly [32]. Therefore, the cells survive and proliferate not only in the peripheral part but also in the center of the scaffold (Fig. 9.3). The early study demonstrated that the dynamic culture was superior to static culture for large scale cell construct cultivation [18]. Moreover, the MSCs can differentiate in to the osteogenic cells by the shear stress created by the perfusion flow. However, the different perfusion rate has a different effect on the cell proliferation and differentiation. It is important to tune the bioreactor in order to achieve a balance between the cell proliferation and differentiation.


Fig. 9.3
Histological section of the cell/TCP composite stained with May-Grünwald Giemsa. After 28 days under three-dimensional dynamic perfusion culture, the MSCs survived and proliferated through the scaffolds (From Xie et al. [18], with permission)
Shear Stress
It is true that the mechanical stress will affect the MSCs differentiation. In the field of bone tissue engineering, shear stress created by the bioreactor will direct the MSCs to differentiate into osteogenic cells. It is reported that the cells in the scaffold increased in rotating wall vessel bioreactor (RWVB) were five times as that in T-flask and spinner flask. And with the stress stimulation inside the fluid in the RWVB, the ALP expression was increased; the formation of mineralized nodules was accelerated [33]. The expression of selected bone marker proteins was also enhanced by the low shear stress under the 3D dynamic flow environment [11, 12]. The shear stress in the rotating bioreactor could be calculated and predicted according to the numerical model [34].
In the perfusion bioreactor, the shear stress produced by the bioreactor has more positive effect on the osteogenic differentiation. Datta et al. [35] found that the inherent osteoinductive potential of bone-like extracellular matrix (ECM) along with fluid shear stresses in the perfusion bioreactor synergistically enhanced the osteodifferentiation of MSCs. Leclerc et al. [36] demonstrated that osteoblastic cells could be successfully cultured inside the microdevices under dynamic conditions and their ALP activity was enhanced. Holtorf et al. [37] and Gomes et al. [38] found that under flow perfusion there was greater scaffold cellularity, alkaline phosphatase activity, osteopontin secretion, and calcium deposition compared with static culture even in the absence of dexamethasone. The results suggested that flow perfusion culture alone induced osteogenic differentiation of rat MSCs and that there was a synergistic effect of enhanced osteogenic differentiation when both chemical stimuli (dexamethasone) and mechanical stimuli (low shear stress) were applied. Further study showed that the effect was dose-dependent. In a study conducted by the Bancroft et al. [39], the marrow stromal osteoblasts were cultured on 3D scaffolds under flow perfusion with different rates of flow for an extended period to permit osteoblast differentiation and significant matrix production and mineralization. They found that with all flow conditions, mineralized matrix production was dramatically increased over statically cultured constructs with the total calcium content of the cultured scaffolds increasing with increasing flow rate. The signal transduction mechanism of shear stress in the osteogenic differentiation is complex and involves multiple extracellular signal-regulated kinases (ERK)-dependent and independent pathways [40].
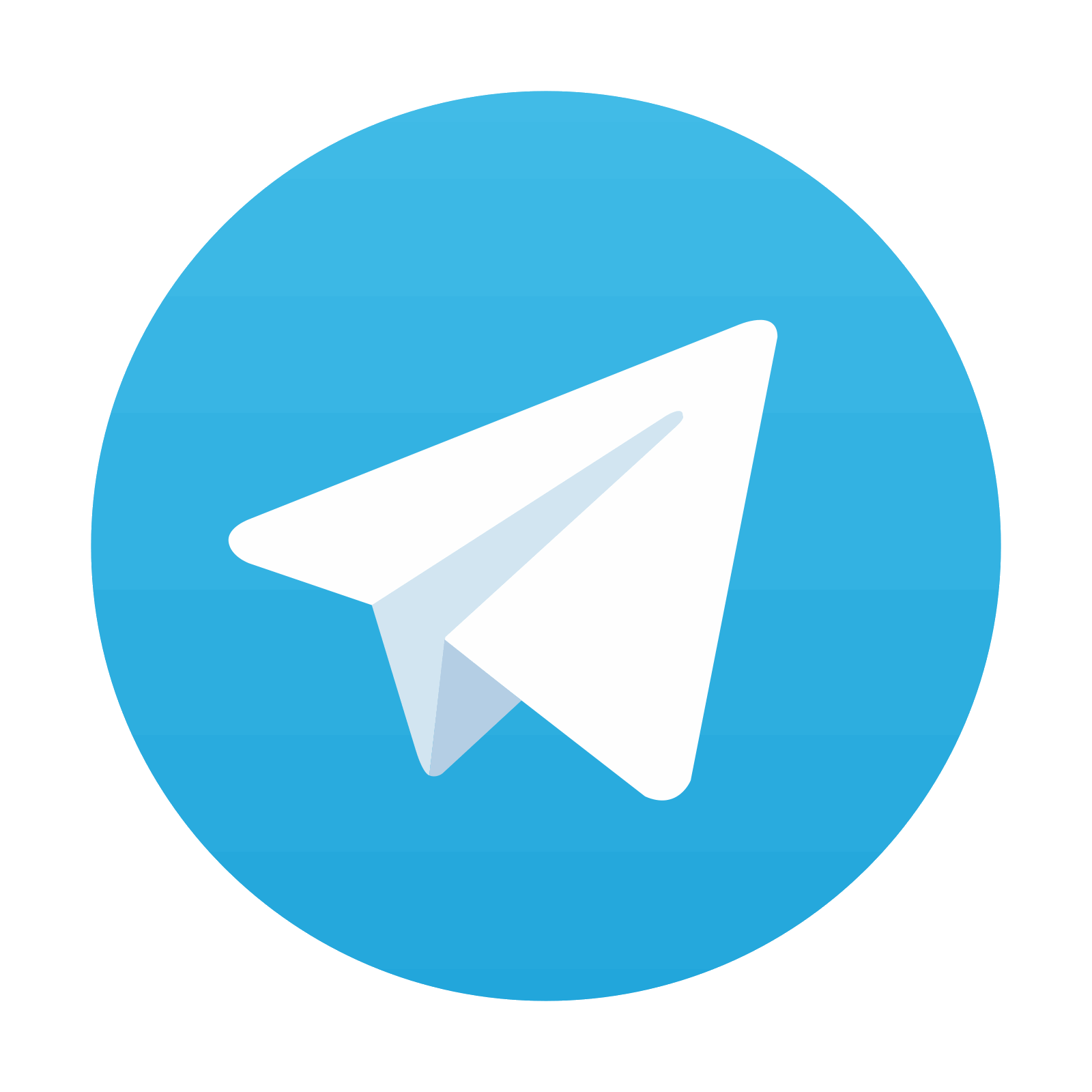
Stay updated, free articles. Join our Telegram channel
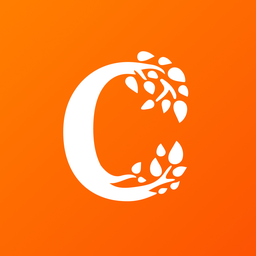
Full access? Get Clinical Tree
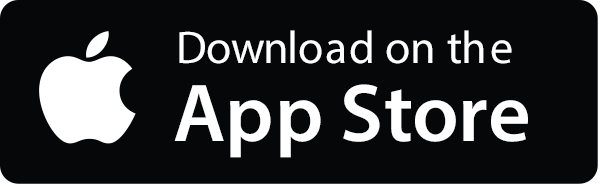
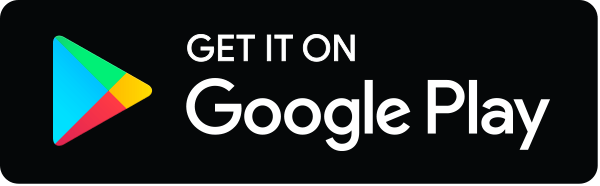