Fig. 42.1
Anterior retroperitoneal approach to the lumbar spine
A total diskectomy is facilitated by mobilizing the disk with interbody distractors and releasing or resecting the posterior annulus and posterior longitudinal ligament (PLL), with possible removal of any posterior osteophytes. The lateral annulus is preserved on both sides. Disk removal is accomplished with standard technique utilizing curettes and rongeurs. It is essential to establish a minimum width of 30–35 mm on the anterior part of the disk to perform the implantation safely. Care is taken to remove the cartilaginous end plates while preserving the bony end plates to minimize the risk of implant subsidence. Attention is paid to complete disk removal retaining only the lateral annulus bilaterally. Special care must be taken with lateral disk resection where any retained disk material can be pushed into the foramen during artificial disk placement. The artificial disk is then placed under AP and lateral fluoroscopic guidance using an individualized combination of sizing, trialing, midline verification, and disk placement.
42.4.2 TDR Devices
Concomitant with the Food and Drug Administration’s (FDA) approval of the Charité artificial disk in October 2004, the era of spinal arthroplasty in the United States was born. Subsequently, the ProDisc-L (2006) received FDA approval. Several more devices have followed (Maverick, Kineflex, FlexiCore, and Activ-L). Cumulatively, these studies have produced a substantial proportion of class one evidence from prospective, randomized studies confirming TDR’s efficacy in the treatment of symptomatic lumbar DDD (Table 42.1).
Table 42.1
FDA-approved artificial disk devices
Artificial disk device | Company | FDA status |
---|---|---|
Charité III | DePuy Spine, Inc. | Approval date: October 2004 |
ProDisc-L | Synthes Spine | Approval date: August 2006 |
42.4.3 Charité (DePuy Spine)
The SB Charité III (DePuy Spine) is one of the most extensively studied intervertebral prostheses to date. The first two designs, SB Charité I and SB Charité II, were implanted in a small number of patients in East Berlin, Germany, and were never made commercially available [32]. These early designs included stainless steel end plates, which were prone to breaking and subsidence. The third-generation and current design (SB Charité III) was first marketed by Waldemar Link outside the United States in 1987, and DePuy Spine acquired the product rights to the Charité artificial disk in 2004. The Charité artificial disk consists of a three-piece design: two cobalt-chrome alloy end plates with spike fixation, with an intervening unconstrained, ultrahigh molecular weight polyethylene sliding core. This offers the theoretical advantage of allowing the spacer to shift dynamically within the disk space during spinal motion, moving posteriorly with flexion and anteriorly in lumbar extension. The primary attachment of the end plates is made possible by six “teeth” on the inferior and superior end plates, and a titanium/calcium hydroxyapatite coating promotes secondary fixation to the vertebral body by allowing bony ingrowth. Although this device was theorized to decrease facet joint arthropathy [32], the actual biomechanical effect of the device in vitro revealed a maximum local loading of facet joints approximately 2.5 times that of intact normal motion segments [26, 32, 33].
In 2009, Guyer et al. published a randomized, multicenter study comparing Charité artificial disk vs. anterior lumbar interbody fusion (ALIF) with BAK cages with a 5-year follow-up. From 160 patients, they did not found statistical differences regarding clinical outcomes between groups and concluded with a noninferiority report compared to ALIF [34].
42.4.4 ProDisc-L Artificial Disk (Synthes)
The ProDisc was designed in the late 1980s by Thierry Marnay, a French orthopedic spine surgeon, and was approved by the US FDA in August 2006 for use at a single lumbar vertebral level. The polyethylene core is secured to the caudal end plate by a modular locking system. Each end plate has a central anchoring keel and two spikes to provide immediate stability. The end plates are coated with a titanium plasmapore surface to enable bony ingrowth for secondary stability. Specially designed instrumentation is used to create a midline groove in the vertebral end plates for implant insertion and to lock the polyethylene core into the caudal end plate. There are two end plate sizes (medium and large), three heights (10, 12, and 14 mm), and two lordosis angles (6° and 11°).
Zigler et al. recently published a prospective, randomized, multicenter study with a 5-year follow-up comparing the ProDisc-L versus circumferential arthrodesis for the treatment of single-level lumbar degenerative disk disease. One hundred sixty-one patients underwent TDRs with the ProDisc-L and 75 patients underwent circumferential fusion. They found that both groups had sustained significant improvement from their preoperative status. No significant differences were found in clinical outcome scales (ODI, SF-36). They concluded that both fusion and TDR are reasonable surgical options [35].
42.4.5 Maverick Artificial Disk (Medtronic: Fig. 42.2)

Fig. 42.2
(a, b) The Maverick artificial disk (With permission and image provided by Medtronic, Inc.) (The Maverick™ disk is not available or approved in the United States)
The Maverick™ artificial disk consists of a cobalt-chrome, two-piece metal-on-metal design with keel fixation and a constrained ball-and-socket design. The center of rotation is fixed and located in the posterior third of the disk space. It is also a semi-constrained device that does not allow for pure translation. These features allow for unloading of the facet joints and reproduction of near-normal force transmission at the operated motion segment. Metal fixation into bone provides immediate stability, and there is no polyethylene wear issue.
Gornet et al. published in 2011 a 2-year follow-up randomized controlled study with 577 patients. Four hundred five patients received the Maverick disk device and 172 anterior interbody fusion (LT-cage). At the last follow-up, the overall success rate for the TDR group was 73.5 %, and for the control group (ALIF), the rate was 55.3 % with a noninferiority conclusion (P < 0.001). Postoperative outcome scales (SF-36 and ODI) also favored the TDR group. There are no differences on adverse events between groups. There were two implant removals in the Maverick group; one was considered to be related to an allergic reaction. Longer follow-up with this two-piece metal-on-metal implant is needed, particularly in light of emerging complications (e.g., pseudotumor formation) with metal-on-metal hip implants. Despite completion of the investigational device exemption study in 2010, the Maverick™ is not currently marketed in the United States due to a patent dispute with another company [36].
42.4.6 Kineflex Artificial Disk (SpinalMotion: Fig. 42.3)

Fig. 42.3
(a–c) The Kineflex artificial disk (With permission and image provided by SpinalMotion, Inc.)
The Kineflex artificial disk consists of cobalt-chrome, metal-on-metal design with a semi-constrained core. The mobile core is seated within a retention ring, and the superior and inferior end plates have multiple serrations, in addition to a central fin allowing for keel fixation and initial stability. On 2011 Kenneth Pettine published a randomized noninferiority trial comparing the Kineflex artificial disk versus the Charité. Sixty-four patients were randomized and followed for 24 months. Eighty-three percent of patients in the Kineflex disk group and 85 % of patients in the Charité group met FDA-defined criteria for clinical success, with no difference between groups (P = 0.802) [37].
42.4.7 FlexiCore Artificial Disk (Stryker Spine: Fig. 42.4)

Fig. 42.4
(a, b) FlexiCore artificial disk (With permission and image provided by Styker)
FlexiCore is a cobalt-chrome metal-on-metal device that is inserted as a single unit. The superior and inferior portions are linked by a captured ball-and-socket joint (constrained domed core). The FlexiCore can be implanted from a straight anterior or an anterolateral direction and offers the ability to manipulate the position of the implant within the intervertebral space. The FlexiCore features unique dome-shaped baseplate surfaces to approximate the concavities of the vertebral body end plates, with several small spikes or “teeth” for immediate bone fixation. These implants are also coated with titanium plasma spray to assist in delayed bony ingrowth for fixation. FDA IDE studies of the FlexiCore disk commenced in 2003 and enrolled and followed over 500 patients. It was also sold outside the United States beginning in 2005. The FlexiCore disk is not currently commercially available [38].
42.4.8 Activ-L Artificial Disk (Aesculap)
The Activ-L Artificial Disk is a next-generation intervertebral disk prostheses consisting of cobalt-chrome end plates with a semi-constrained, polyethylene core (ball-and-socket design). There are modular end plates available, in both spike and keel fixation. Low implant height (with a total height of 8.5 mm) may reduce disk space over-distraction. The convex-shaped end plate allows an ideal contact surface with the concave vertebral end plates. Importantly, the device allows for rotation and controlled anteroposterior translation of the polyethylene core, similar to the native IAR. The combination of rotation and translation may serve to unload the facets joints, thereby preventing implant-related facet arthrosis. An FDA trial started in 2007, enrollment was completed and currently it is in the final stages of data collection and submission [38].
There are also other devices that are currently on trials, such as the NuVasive XL TDR® (NuVasive – Fig. 42.5). It has metallic design that is implanted from the patient’s side, with a similar approach to extreme lateral interbody fusion (XLIF) cages. An FDA trial started in 2009. The NuVasive XL TDR® device is not approved in the United States and limited by US law to investigational use.


Fig. 42.5
(a–d) XL TDR (With permission and image provided by NuVasive, Inc.) (The NuVasive XL TDR® device is not approved in the United States. Caution – investigational device. Limited by US law to investigational use)
The Freedom Lumbar Disk (AxioMed – Fig. 42.6) is made of a silicone polycarbonate-urethane polymer core that is bonded between the two metal end plates to allow controlled motion and enable shock absorption capability. The device has been used in Europe, and FDA trials began in 2008. The Triumph (Fig. 42.7) consists of two metallic end plates with a geometry allowing it to be inserted through a posterolateral approach. An IDE study started in 2007; it is still ongoing but no longer recruiting.



Fig. 42.6
Freedom TDR (With permission and image provided by AxioMed)

Fig. 42.7
Triumph TDR is an investigation device in the United States (With permission and image provided by Globus Medical, Inc.)
42.5 Biomechanics of TDR Prostheses
42.5.1 Intervertebral Disk and Disk Replacement Center of Rotation
The center of rotation of the lumbar spinal motion segment does not move about a single fixed axis of rotation, but rather an elliptical locus of instantaneous axes of rotation, termed the centrode [39–42]. The centrode is located within the posterior half of the disk space in the normal intact spine. In early degenerative disk disease, the centrode lengthens, and in moderate disk degeneration, the centrode migrates caudally. Axial loading does not appear to influence the centrode dimensions or position.
Using an in vitro human cadaveric model, the multidirectional flexibility properties and COR of TDR (SB Charité) were determined and compared to conventional threaded fusion cages (e.g., BAK cages) and BAK cages augmented with transpedicular screw/rod fixation for single-level spinal instrumentation [43, 44]. The SB Charité prosthesis was associated with an increase in axial rotation by 44 % compared to the intact lumbar spine, whereas the BAK and anteroposterior reconstructions decreased range of motion by 29 % and 80 %, respectively. Flexion-extension range of motion was slightly increased for the SB Charité (3 %) versus the intact disk, whereas this was decreased in the BAK and anteroposterior stabilization groups (BAK = 57 %, anteroposterior = 93 %) when compared to the intact and SB Charité conditions. Based on flexion-extension radiographs, the intervertebral centers of rotation were in the posterior one-third of the operative intervertebral disk only for the SB Charité device and intact spine condition, with definitive evidence of physiologic intervertebral translation. Therefore, the TDR device preserved the kinematic properties and normal mapping of segmental motion at the operative and adjacent intervertebral disk levels, as compared to interbody instrumentation, with or without transpedicular screw/rod fixation.
42.5.2 Load Sharing After Lumbar Disk Arthroplasty
Using a finite element model, Dooris et al. [45] implanted a ball-and-cup-type artificial disk via an anterior approach and compared the data with in vitro data. Both small and large annulotomies were performed, and the implant was placed either anteriorly or posteriorly within the disk space. The restoration of an intact ALL was also assessed. Models were subjected to either an axial compression force alone or a combination of flexion-extension moment with axial preload. In this model, the facet loads were found to be more sensitive to the anteroposterior location of the artificial disk than to the degree of annulotomy. Under pure axial compression, implanted models with an anteriorly placed artificial disk exhibited facet loads 2.5 times greater than loads observed with the intact model, whereas posteriorly implanted models demonstrated no facet loads in compression. Implanted models with a posteriorly placed disk also exhibited greater flexibility than the intact and implanted models with anteriorly placed disks. The restoration of the anterior longitudinal ligament resulted in reduced pedicle stresses, facet loads, and extension-rotation to near normal. This study suggested that by altering the placement of the artificial disk in the anteroposterior direction, the motion segment flexural stiffness and posterior load sharing may be modulated [45].
Biomechanical comparisons have also been performed between unconstrained and constrained lumbar artificial disk designs. These studies have revealed differences in facet loading and implant stresses (e.g., polyethylene core) [46]. An unconstrained artificial disk design is less sensitive to placement and unloads the facet joints, compared with a constrained design. The decreased core stress may result in a reduced potential for wear in an unconstrained prosthesis, which may potentially increase the functional longevity of the device.
42.5.3 Load-Displacement Curve and Motion Patterns of TDR Devices
The pattern of intervertebral load-displacement curves for Charité TDR-implanted motion segments has been studied by O’Leary et al. [47]. The prosthesis component motion patterns (PCMP) were divided into four types: PCMP1, angular motion predominantly between the upper end plate and core, with little or no visible core translation; PCMP2, lift-off of upper prosthesis end plate from core or of core from lower end plate; PCMP3, core entrapment, resulting in a locked core over a portion of the range of motion; and PCMP4, angular motion between both the upper and lower end plates and core, with visible core translation [47].
A gradually changing motion pattern was observed in normal lumbar segments, while the TDR-implanted segments displayed regions of both relatively small and large angular changes with gradual moment application. The disruption of the ALL and the anterior annulus during the insertion of the Charité TDR removes the biomechanical constraints imposed by these structures. As a result, a larger angular travel was observed in the absence of physiologic compressive preload for the same applied moment in TDR motion segments, compared with the intact motion segments. Under a compressive preload, entrapment and locking of the polyethylene core occurred over a portion of the sagittal plane motion – as reflected by the relatively flat portion of the load-displacement curves in the presence of a preload (Fig. 42.8). Once the core was released, a large angular change occurred, reflected by a sharp rise of the load-displacement curves (Fig. 42.8). The predominant angular motion within the prosthesis occurred between the upper end plate and the polyethylene core.


Fig. 42.8
Load angular displacement curves of intact lumbar spine and Charité TDR device implanted at the (a) L5–S1 and (b) L4–L5 levels in human lumbar spine. (a) Prosthesis motion pattern demonstrating core entrapment. A locked core is observed over a portion of the range of motion, with large angular change occurring with core release. (b) Prosthesis motion pattern with angular motion between both the upper and lower end plates and core, with visible core translation (Figure kindly provided by Dr. Avinash Patwardhan)
The effects that these TDR motion patterns may have on the long-term outcome, as well as on load sharing at the implanted level and polyethylene core wear, currently remain unknown. However, the nonuniform motion could potentially influence the wear of the implant. Other movement patterns, such as angular motion at one articulation with only a small amount of core translation, may also potentially influence load sharing within the implanted segment and at adjacent levels. Based on biomechanical studies, it may be concluded that several factors are likely to affect the function of the non- or semi-constrained TDR implants, including implant placement and orientation, intraoperative changes in lordosis, and the magnitude of physiologic compressive preload [47].
42.5.4 Adjacent-Segment Degeneration
Accelerated adjacent-segment degeneration is one of the most important morbidities associated with solid spinal fusion. It is for this reason that there is such a significant development of non-fusion motion preservation devices. Short-term results for the lumbar disk arthroplasty devices are encouraging. Long-term results, however, are not yet available. Appropriately designed biomechanical studies of adjacent-level degeneration have provided insight into the potential success or failure of an implant with respect to motion preservation and adjacent-level degeneration. Although many biomechanical studies are available, the results have large variation and are conflicting, mostly due to the use of inappropriate and ill-defined methodologies. A relatively new testing method designed to study spinal adjacent-level effects, the hybrid test method, uses unconstrained pure moments to provide rotation input for multidirectional testing. The hybrid test method has four steps: (1) intact spine specimen with entire mobile region is used to measure various biomechanical parameters, e.g., disk pressures, ligament strains, and facet loads; (2) appropriate unconstrained pure moment is applied to the intact specimen and total range of motion is determined; (3) unconstrained pure moment is applied to the spinal construct (specimen with an implant) until the total range of motion of the construct equals that of the intact; and (4) statistical comparison of the biomechanical parameters between the construct and intact then allows for comparison of the adjacent-level effects [48–51]. Using a hybrid test method with a whole lumbar spine specimen (T12–S1), multidirectional adjacent-level effects due to implantation of one- and two-level ProDisc-L device were evaluated and compared to simulated one- and two-level arthrodesis. The single-level lumbar ProDisc-L preserved rotations at the non-operated levels but showed increased rotations at the operated level in both lateral bending and torsion. Conversely, two-level simulated fusion decreased the rotations at the fusion site and produced increased adjacent-level rotations in all directions, compared with lumbar arthroplasty devices. Therefore, it may be concluded that rotation lost/gained at the operated level is redistributed over the remainder levels. ProDisc-L implantation produced only small adjacent-level effects in comparison with significant effects produced by spinal fusion. Importantly, the study revealed that the adjacent-level effects were not confined to the adjacent levels alone but were seen throughout the entire specimen (i.e., whole lumbar spine specimen, T12–S1).
Adjacent-level biomechanics after multilevel disk arthroplasty in cadaveric lumbar spines have been evaluated by comparing operative- and adjacent-segment range of motion and intradiscal pressures [52]. The study compared two-level disk arthroplasty versus circumferential arthrodesis using anterior interbody cages and pedicle screws. A kinematics assessment revealed that segmental motion distributed over L2–S1 was preserved in the arthroplasty group but was significantly altered after circumferential fusion. After arthrodesis, adjacent-level range of motion and intradiscal pressures were increased proximally and distally under loading modalities of flexion-extension, axial rotation, and lateral bending. In contrast, there was no significant difference in either the range of motion or intradiscal pressures at adjacent levels between intact control and disk arthroplasty groups.
Harrop et al. [53] have performed a systematic review of the published incidence of radiographic adjacent-segment degeneration and symptomatic adjacent-segment disease after arthrodesis or total disk replacement. Their data supported the use of arthroplasty to reduce adjacent-level disk degeneration and disease, compared to arthrodesis. In the study, 34 % of patients undergoing arthrodesis and 9 % of patients in the total disk replacement group developed adjacent-level degeneration. Increased risk of adjacent-level degeneration was associated with older patients, arthrodesis, and longer follow-up [30, 53]. The risk of adjacent-segment failure appears to be higher for patients in whom lumbar fusion with rigid instrumentation is performed to treat degenerative instability, and the risk seem to be particularly high in postmenopausal women [54]. Adjacent-segment disease developed in 14 % arthrodesis patients compared to 1 % of arthroplasty patients. These results suggested a correlation between fusion and the development of adjacent-level degeneration, compared to arthroplasty. However, the association was dampened by the influence of patient age. Nevertheless, a strong correlation existed between spinal fusion and adjacent-level disease, compared to arthroplasty.
Another systematic review done by Wang et al. compared the incidence of adjacent-segment disease of two randomized controlled trials (Berg et al. and Guyer et al.), after a 2-year follow-up. The risk of clinical adjacent-segment disease (treated surgically) was 1.2 % (2/170) on the TDR group versus 7.0 % (8/115) on the fusion group. Another more recent study done by Zigler et al. published a randomized controlled trial with a 5-year follow-up, comparing the ProDisc-L versus circumferential fusion. Three (1.9 %) of 161 of the TDR group and 3 (4 %) of 75 fusion group required surgery at the adjacent level [38].
The aforementioned studies are challenged, however, by existing data that suggests that incidence of adjacent-segment disease is no greater in fused spines than in spines that did not undergo fusion. Furthermore, the maintenance of a lordotic posture appears to be critical for the prevention of adjacent-segment degeneration and disease [43, 55–68].
42.5.5 Motion Preservation
The preservation of segmental mobility represents a potential advantage of lumbar disk arthroplasty. One of the goals of lumbar arthroplasty is to restore and maintain mobility and to protect adjacent levels from abnormal motion, which may be a factor in transition syndrome following arthrodesis. The restoration of disk motion may preserve lumbar facet structure and function and prevent early degeneration. Results from clinical trials of disk replacement, however, do not yet have sufficient follow-up to determine whether the cascade of facet joint arthropathy is, in fact, halted or retarded. The mobility observed with TDR appears to be approximately 6° in flexion-extension at the L5–S1 level and 8° at L4–L5 [69]. Mobility in lateral inclination has also been analyzed and was found to be approximately 3–4°. Clinical and radiologic outcomes with a minimum follow-up of 10 years have been reported for the Charité artificial disk [69]. A total of 107 patients implanted with the Charité prosthesis through a standard anterior retroperitoneal approach (147 prostheses implanted, 54 one-level procedures, 45 two-level procedures, and 1 three-level procedure) were evaluated. Clinically, 62 % had an excellent outcome, 28 % had a good outcome, and 10 % had a poor outcome. Mean flexion-extension motion was 10.3° for all levels (12.0° at L3–L4, 9.6° at L4–L5, 9.2° at L5–S1). The mean lateral motion was 5.4°. In the sagittal plane, 6.1 % of the devices were anterior of geometric center, 34.0 % were centered, and 59.9 % were posterior of center. In the frontal plane, 75 % were centered, and 25 % were lateral of center. No subluxation of the prostheses and no cases of spontaneous arthrodesis were identified. Thus, with a minimum follow-up of 10 years, the Charité artificial disk demonstrated excellent flexion-extension and lateral range of motion with no significant complications [69].
The distribution of in vivo and in vitro range of motion (ROM) following single-level arthroplasty with the SB Charité III artificial disk has been compared with posterolateral fusion [70]. In this study, in vitro ROM in flexion-extension at the implanted and adjacent levels was measured, and the results were compared with in vivo, 2-year postoperative radiographic ROM evaluations. The results showed that single-level arthroplasty appeared to replicate the normal distribution of motion of the intact spine [17, 70].
A human cadaveric biomechanical study was performed to measure the facet forces and the IAR for different spinal positions under simulated weight-bearing conditions before and after total disk replacement at L5–S1 using semi-constrained (3° of freedom; ProDisc) and unconstrained (5° of freedom; Charité) articulated implants. With the ProDisc, the facets were partially unloaded, though the IAR did not match the fixed geometric center of the UHMWPE core, suggesting that joint surface incongruence is developed during movement. With the Charité, the IAR was less variable, yet the facet forces tended to increase particularly during lateral bending. These results highlight the important role that the facets play in guiding movement and that implant constraint influences facet-implant synergy. Rotational hypermobility and excessive torsional forces are potentially undesirable effects. Such hypermobility may induce excessive loads on the posterior facets, promoting facet arthrosis and nerve root impingement. Moreover, the posterior elements in many patients with mechanical LBP are not fully intact, leading to further events of the degenerative cascade (e.g., disk degeneration, facet arthrosis) and subsequently producing pain.
The biomechanics of the lumbar spine treated either by fusion or total disk replacement (TDR) have been compared under severe loading conditions [71]. A three-dimensional model of a two-level ligamentous lumbar segment was created and simulated through static analyses with the finite element method. The analysis predicted that mobility after arthrodesis on the upper level was reduced in all rotational degrees of freedom by an average of approximately 44 %, relative to healthy normal disks. Conversely, the mobility after TDR on the upper level was increased in all rotational degrees of freedom by 52 %. The level implanted with the artificial disk showed excessive ligament tensions, high facet pressures, and a high risk of instability. The mobility and the stresses in the level adjacent to the arthroplasty were also increased. This model for an implanted artificial disk showed a greater risk of instability and further degeneration than predicted for the arthrodesis model [72].
42.5.6 Stability of the Implant-Bone Interface
The stability of an implanted lumbar arthroplasty device may be conceptualized into three categories: (1) short-term or immediate stability, (2) intermediate stability, and (3) long-term stability. The anchoring to the vertebral end plates via spikes or midline keel is considered important for immediate implant stability. The original SB Charité prosthesis was not solidly anchored, and this may have been associated with early expulsion of the device [32]. Several device end plates have been modified with a porous titanium and electrochemically bonded calcium hydroxyapatite coating, which allow for bony ingrowth into the end plate, providing intermediate or secondary stability with a potentially reduced risk of device expulsion [73]. Long-term stability may be defined as the cellular and microscopic changes of bony ingrowth and integration at the bone interfaces.
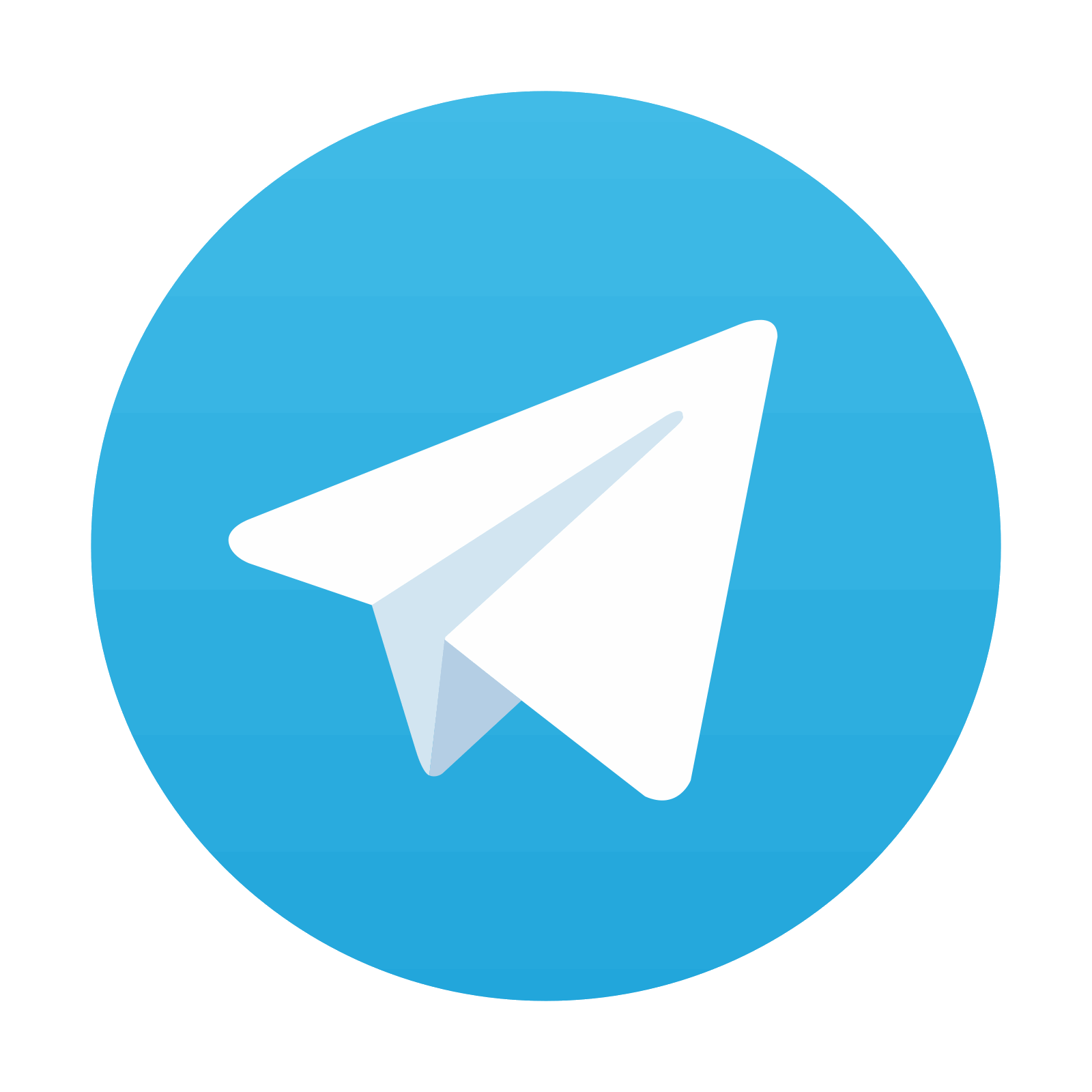
Stay updated, free articles. Join our Telegram channel
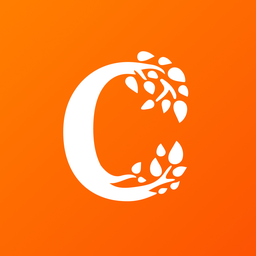
Full access? Get Clinical Tree
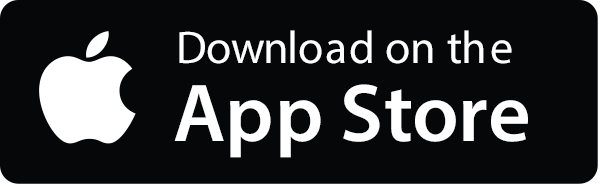
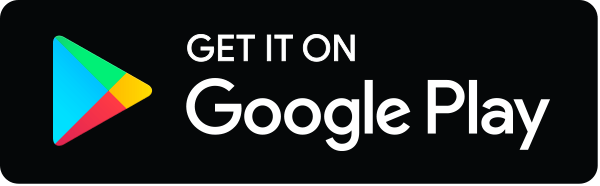