© Springer Science+Business Media New York 2015
Narayan Yoganandan, Alan M. Nahum, John W. Melvin and The Medical College of Wisconsin Inc on behalf of Narayan Yoganandan (eds.)Accidental Injury10.1007/978-1-4939-1732-7_99. Biomechanics of Brain Injury: A Historical Perspective
(1)
Department of Neurosurgery, Medical College of Wisconsin, Milwaukee, WI, USA
Deceased
Abstract
The brain may be the organ most critical to protect from trauma, because anatomic injuries to its structures are currently nonreversible, and consequences of injury can be devastating. The experimental study of brain-injury mechanisms is unparalleled, because effects of trauma to the organs responsible for control and function of the body are the objects of the study. Injury of the central nervous system results not only from local primary effects, but from effects on physiologic homeostasis that may lead to secondary injury. The brain controls the flow of information, including autonomic control as well as sensory perception and motor function. The brain is the source of intentional actions, and it functions in time to store, retrieve, and process information. The state of self-awareness or consciousness is the highest level of brain function in man.
This chapter addresses the historical state of knowledge in the biomechanics of brain injury. It is organized to provide a summary of head and brain anatomy and of clinical head-injury issues, followed by sections on experimental brain-injury models and bio-mechanical mechanisms of brain-injury and head-injury criteria. It serves as a preface to the following chapter on the future of brain injury biomechanics.
9.1 Anatomy of the Head
This section is a brief summary of head anatomy, starting with the scalp and skull, followed by more detailed descriptions of the structures internal to the skull. It is intended to provide a basic description for use in the following sections of the chapter. More complete descriptions can be found in standard anatomy texts.
9.1.1 Scalp
The scalp is 5–7 mm (0.20–0.28 in.) thick and consists of three layers: the hair-bearing skin (cutaneous layer), a subcutaneous connective-tissue layer, and a muscle and fascial layer. Beneath the scalp there is a loose connective-tissue layer plus the fibrous membrane that covers bone (periosteum). The thickness, firmness, and mobility of the outer three layers of scalp as well as the rounded contour of the cranium function as protective features. When a traction force is applied to the scalp, its outer three layers moved together as one.
9.1.2 Skull
The skull is the most complex structure of the skeleton. This bony network is neatly molded around and fitted to the brain, eyes, ears, nose, and teeth. The thickness of the skull varies between 4 and 7 mm (0.16 and 0.28 in.) to snugly accommodate and provide protection to these components. The skull is composed of eight bones that form the brain case, 14 bones that form the face, as well the teeth. Excluding the face, the cranial vault is formed by the ethmoid, sphenoid, frontal, two temporal, two parietal, and occipital bones. The inner surface of the cranial vault is concave and relatively smooth. The base of the braincase is an irregular plate of bone containing depressions and ridges plus small holes (foramen) for arteries, veins, and nerves, as well as the large hold (the foramen magnum) that is the transition area between the spinal cord and the brainstem.
9.1.3 Meninges
Three membranes know as the meninges protect and support the brain and spinal cord. One function of the meninges is to isolate the brain and spinal cord from the surrounding bones. The meninges consist primarily of connective tissue, and they also form part of the walls of blood vessels and the sheaths of nerves as they enter the brain and as they emerge from the skull.
The meninges consist of three layers: the dura mater, the arachnoid, and the pia mater. The dura mater is a tough, fibrous membrane that surrounds the spinal cord and, in the skull, is divided into two layers. The outer cranial, or periosteal layer, lines the inner bony surface of the calvarium. The inner layer, or meningeal layer, covers the brain. In the braincase, the two layers of dura mater are fused except where they separate to form venous sinuses, which drain blood from the brain. Folds of the meningeal layer form the falx cerebri, which projects into the longitudinal fissure between the right and left cerebral hemispheres; and the tentorium cerebelli, a shelf on which the posterior cerebral hemispheres are supported.
The arachnoid mater is a delicate spider-web-like membrane that occupies the narrow subdural space. The pia mater is a thin membrane of fine connective tissue invested with numerous small blood vessels. It is separated from the arachnoid by the subarachnoid space. The pia mater covers the surface of the brain, dipping well into its fissures.
The subarachnoid space and the ventricles of the brain are filled with a colorless fluid (cerebrospinal fluid, or CSF), that provides some nutrients for the brain and cushions the brain from mechanical shock. Cerebrospinal fluid is formed in the lateral and third ventricles by the choroid plexus and passes via the cerebral aqueduct into the fourth ventricle. From this site the fluid circulates in the sub-arachnoid spaces surrounding both the brain and the spinal cord. The majority of the CSF is passively returned to the venous system via the arachnoid villi. The specific gravity of cerebrospinal fluid is about 1.008 in the adult, which is approximately that of blood plasma. About 140 ml of CSF constantly circulates and surrounds the brain on all sides, so it serves as a buffer and helps to support the brain’s weight. Since the subarachnoid space of the brain is directly continuous with that of the spinal cord, the spinal cord is suspended in a tube of CSF. For normal movement, a shrinkage or expansion of the brain is quickly balanced by an increase or decrease of CSF.
9.1.4 Central Nervous System
The central nervous system (CNS) consists of the brain and the spinal cord. At a microscopic level, the CNS is primarily a network of neurons and supportive tissue functionally arranged into areas that are gray or white in color. Gray matter is composed primarily of nerve-cell bodies concentrated in locations on the surface of the brain and deep within the brain; white matter is composed of myelinated nerve-cell processes (axons) that largely form tracts to connect parts of the central nervous system to each other.
The brain can be divided structurally and functionally into five parts: cerebrum, cerebellum, midbrain, pons and medulla oblongata. In addition, it has four ventricles (CSF cisterns with exits), three membranes (meninges), two glands (pituitary and pineal), 12 pairs of cranial nerves, and the cranial arteries and veins. The average length of the brain is about 165 mm (6.5 in.), and its greatest transverse diameter is about 140 mm (5.5 in.). Because of size differences, its average weight is 1.36 kg (3.0 lb) for the male and a little less for the female. The specific gravity of the brain averages 1.036, and it is gelatinous in consistency. The brain constitutes 98 % of the weight of the central nervous system and represents about 2 % of the weight of the body.
9.1.5 Cerebrum
The cerebrum makes up seven-eighths of the brain and is divided into right and left cerebral hemispheres. These are incompletely separated by a deep midline cleft called the longitudinal cerebral fissure. The falx cerebelli process projects downward into this fissure. Beneath the longitudinal cerebral fissure, the two cerebral hemispheres are connected by a mass of white matter called the corpus callosum. Within each cerebral hemisphere is a cistern for cerebrospinal fluid called the lateral ventricle. The surface of each hemisphere is composed of gray matter and referred to as the cerebral cortex. In man, the cerebral cortex is arranged in a series of folds or convolutions. The ridge of the fold is referred to as a gyrus whereas the valley of the fold is called a sulcus. Each cerebral hemisphere is further subdivided into four lobes by fissures, each lobe being named by its association to the nearest cranial bone. Thus, the four lobes are the frontal, parietal, temporal, and occipital lobes.
The interior of each cerebral hemisphere is composed of white matter arranged in tracts that serve to connect one part of cerebral hemisphere with another, to connect the cerebral hemispheres to each other, and to connect the cerebral hemispheres to the other parts of the central nervous system. In addition, within these interior areas of white matter are concentrations of gray matter called nuclei.
9.1.6 Midbrain
The midbrain connects the cerebral hemispheres above to the pons below. Anteriorly the midbrain is composed of two stalks that consist of fibers passing to and from the cerebral hemispheres above. The midbrain also contains gray matter nuclei. Within the midbrain is a narrow canal, the cerebral aqueduct, which connects to the third ventricle above the fourth ventricle below.
9.1.7 Pons
The pons lies below the midbrain, in front of the cerebellum, and above the medulla oblongata. It is composed of white matter nerve fibers connecting the cerebellar hemispheres. Lying deep within its white matter are areas of gray matter that are nuclei for some of the cranial nerves.
9.1.8 Medulla Oblongata
The medulla oblongata appears continuous with the pons above and the spinal cord below. In the lower part of the medulla oblongata, motor fibers cross from one side to the other so that fibers from the right cerebral cortex pass to the left side of the body. Some sensory fibers passing upward toward the cerebral cortex also cross from one side to the other in the medulla oblongata. The medulla oblongata also contains areas of gray matter within its white matter. These are nuclei for cranial nerves and relay stations for sensory fibers passing upward from the spinal cord.
9.1.9 Cerebellum
The cerebellum lies behind the pons and the medulla oblongata. Its two hemispheres are joined at the midline by a narrow, striplike structure called the vermis. The outer cortex of the cerebellar hemispheres is gray matter; the inner cortex is white matter. The outer surface of the cerebellum forms into narrow folds separated by deep fissures. Nerve fibers enter the cerebellum in three pairs of stalks that connect the cerebellar hemispheres to the midbrain, pons, and medulla oblongata.
9.2 Traumatic Brain Injury from Clinical Experience
9.2.1 Introduction
Data compiled by the Department of Health and Human Services (1989) indicate that someone receives a head injury every 15 s in the United States. This places the total number of injuries at over two million per year with half a million severe enough to require hospital admission. This study further indicates that 75,000–100,000 die each year as a result of traumatic brain injury, and it is the leading cause of death and disability in children and young adults.
9.2.2 Types of Brain Injuries
Clinically brain injuries can be classified in two broad categories: diffuse injuries and focal injuries. The diffuse injuries consist of brain swelling, concussion, and diffuse axonal injury (DAI). The focal injuries consist of epidural hematomas (EDH), subdural hematomas (SDH), intracerebral hematomas (ICH), and contusions (coup and contrecoup). Studies have attempted to describe the incidence and sequelae of the above brain injuries [1–3]. The results of these studies vary somewhat, however, mainly due to the criterion of selection of the patients in the studies. For example, the patients in the Chapon, et al. study were all critically injured with immediate unconsciousness without a lucid interval after accidents involving automobiles (occupants and pedestrian) and two-wheelers [1]. Only 7 % of their sample contained injuries from falls and sports. Gennarelli’s study contained 48 % patients whose injuries were due to falls and assaults while the other 52 % were from automobile accidents (occupants and pedestrians) and data indicated that the average injury was serious to severe [2]. This difference in the population considered may explain the different results in the two series, since Gennarelli’s study shows a marked difference in the type of brain injuries sustained by automobile accident victims as compared to assault and fall victims. In this study, it was found that three out of four automobile/pedestrian brain injuries were of the diffuse type, and one out of four was of the focal type. The assault and fall victims had one out of four diffuse injuries and three out of four focal injuries.
Gennarelli’s study points out that acute subdural hematoma and diffuse axonal injury were the two most important causes of death. These two lesions together accounted for more head injury deaths than all other lesions combined. The injuries most often associated with a good or moderate recovery were cerebral concussions and cortical contusion.
9.2.3 Diffuse Injuries
Diffuse brain injuries form a spectrum of injuries ranging from mild concussion to diffuse white matter injuries. In the mildest forms, there is mainly physiological disruption of brain function and, at the most severe end, physiological and anatomical disruptions of the brain occur.
Mild concussion does not involve loss of consciousness. Confusion, disorientation, and a brief duration of post-traumatic and retrograde amnesia may be present. This is the most common form of diffuse brain injury, it is completely reversible, and due to its mildness it may not be brought to medical attention. According to Scott, minor concussions form approximately 10 % of all minor-to-serious injuries involving the brain, skull, and spinal column [4].
The classical cerebral concussion involves the immediate loss of consciousness following injury. Clinically, the loss of consciousness should be less than 24 h and is reversible. Post-traumatic and retrograde amnesia is present, and the duration of amnesia is a good indicator of the severity of concussion. Thirty-six percent of these cases involve no lesions of the brain. The remainder may be associated with cortical contusions (10 %), vault fracture (10 %), basilar fracture (7 %), depressed fracture (3 %), and multiple lesions (36 %) [5]. Hence, the clinical outcome of patients with this type of symptom depends on the associated brain injuries. In general, 95 % of the patients have good recovery at the end of 1 month. Close to 2 % of the patients might have severe deficit, and 2 % might have moderate deficit [5].
The injury considered to be the transition between pure physiological dysfunction of the brain to anatomical disruption of the brain generally involves immediate loss of consciousness lasting for over 24 h. This injury is also called diffuse brain injury. It involves occasional decerebrate posturing, amnesia lasting for days, mild-to-moderate memory deficit, and mild motor deficits. At the end of 1 month, only 21 % of these cases have good recovery. Fifty percent of the cases end up with moderate to severe deficits, 21 % of the cases have vegetative survival, and 7 % are fatal [5]. The incidence of diffuse injury among severely injured patients is 13 % [5] to 22 % [1].
Diffuse axonal injury (DAI) is associated with mechanical disruption of many axons in the cerebral hemispheres and subcortical white matter. Axonal disruptions extend below the midbrain and into the brainstem to a variable degree [6]. These are differentiated from the less-severe diffuse injuries by the presence and persistence of abnormal brainstem signs. Microscopic examination of the brain discloses axonal tearing throughout the white matter of both cerebral hemispheres. It also involves degeneration of long white-matter tracts extending into the brainstem. High-resolution CT scans may show small hemorrhages of the corpus callosum, superior cerebellar peduncle, or periventricular region. These hemorrhages are quite small and may often be missed on CT scans.
Diffuse axonal injury involves immediate loss of consciousness lasting for days to weeks. Decerebrate posturing, with severe memory and motor deficits, is present. Post-traumatic amnesia may last for weeks. At the end of 1 month, 55 % of the patients are likely to have died, 3 % might have vegetative survival, and 9 % would have severe deficit [5].
Brain swelling, or an increase in intravascular blood within the brain, may be superimposed on diffuse brain injuries, adding to the effects of the primary injury by increased intracranial pressure [5]. Penn and Clasen report from 4 % to 16 % of all head-injured patients and 28 % of pediatric head-injured patients have brain swelling [7]. Tarriere has reported the incidence of edema in 21 % of CT-scanned head-injured subjects [3]. It should be noted that in general brain swelling and edema are not the same but are often used interchangeably in literature. Cerebral edema is a special situation in which the brain substance is expanded because of an increase in tissue fluid [7]. The course of treatment for the two may be different. According to Penn and Clasen, the mortality rate due to brain swelling among adults is 33–50 % and 6 % in children [7].
9.2.4 Focal Injuries
Acute subdural hematoma (ASDH) has three sources: direct lacerations of cortical veins and arteries by penetrating wounds, large-contusion bleeding into the subdural space, and tearing of veins that bridge the subdural space as they travel from the brain’s surface to the various dural sinuses. The last mechanism is the most common in the production of ASHD [5]. Gennarelli and Thibault report a high incidence (30 %) of ASDH among severely head-injured patients, with a 60 % mortality rate. From various earlier studies, Cooper reports the incidence rates of ASDH to be between 5 % and 13 % of all severe head injuries [8]. According to Cooper, acute subdural hematomas generally coexist with severe injury to the cerebral parenchyma, leading to poorer outcome when compared to chronic subdural and extradural hematomas that generally do not coexist with injuries to the cerebral parenchyma. The mortality rate in most studies is greater than 30 % and greater than 50 % in some.
Extradural hematoma (EDH) is an infrequently occurring sequel to head trauma (0.2–6 %). It occurs as a result of trauma to the skull and the underlying meningeal vessels and is not due to brain injury [8]. Usually skull fracture is found, but EDH may occur in the absence of fracture. From 50 % to 68 % of the patients have no significant intracranial pathology [8]. The remainder of the patients may have subdural hematoma and cerebral contusions associated with the EDH. These associated lesions influence the outcome of the EDH. The mortality rate from various studies ranges from 15 % to 43 %. This rate is greatly influenced by age, presence of intradural lesions, the time from injury to the appearance of symptoms, level of consciousness, and neurological deficit [8].
Cerebral contusion is the most frequently found lesion following head injury. It consists of heterogeneous areas of necrosis, pulping, infarction, hemorrhage, and edema. Some studies have shown the occurrence of contusions in 89 % of the brains examined post-mortem [8]. CT-scan studies have shown incidence rates from 21 % to 40 %. A recent CT-scan study by Tarriere shows an incidence of 31 % for contusions alone and 55 % associated with other lesions [3]. In contrast, Gennarelli’s study [5] shows an incidence of contusions in only 13 % of the patients studied.
Contusions generally occur at the site of impact (coup contusions) and at remote sites from the impact (contrecoup contusions). The contrecoup lesions are more significant than the coup lesions [8]. They occur predominantly at the frontal and temporal poles, which are impacted against the irregular bony floor of the frontal and middle fossae. Contusions of the corpus callosum and basal ganglia have also been reported [8]. Contusions are most often multiple and are frequently associated with other lesions (cerebral hemorrhage, SDH and EDH). Contusions are frequently associated with skull fracture (60–80 %). In some cases they appear to be more severe when a fracture is present than when it is absent [8]. Mortality from contusions is reported to range from 25 % to 60 % [8]. Adults over 50 years of age fare worse than children. Patients in coma have a generally poor outcome.
Intracerebral hematomas (ICH) are well-defined homogeneous collections of blood within the cerebral parenchyma and can be distinguished from hemorrhagic contusions (mixture of blood and contused and edematous cerebral parenchyma) by CT scans. They are most commonly caused by sudden acceleration/deceleration of the head. Other causes are penetrating wounds and blows to the head producing depressed fractures below which ICH develop. Hemorrhages begin superficially and extend deeply into the white matter. In one-third of the cases they extend as far as the lateral ventricle. Some cases of the hematomas extending into the corpus callosum and the brainstem have been reported [8].
According to Cooper, the incidence of ICH has been underestimated in the past. With the advent of the CT scan, better estimates are now available [8]. Recent studies show the incidence to be between 4 % and 23 %. Gennarelli shows an incidence rate of 4 % and Chapon et al. (1983) show an incidence rate of 8 % in severely injured patients [1, 5].
Depending on the study, the mortality for traumatic ICH has been as high as 72 % and as low as 6 %. The outcome is affected considerably by the presence or absence of consciousness of the patient [8].
9.2.5 Skull Fractures
Clinically the presence or absence of linear skull fracture does not have much significance for the course of brain injury, although the subject is still controversial. This controversy continues because some studies show that, when dangerous complications develop after an initial mild injury, they are associated with skull fracture [9]. According to Cooper, failure to detect fractures would have little effect on the management of the patient [10]. Cooper further cites a study in which half the patients with extradural hematomas had skull fracture and half did not. Another study showed the occurrence of subdural hematomas in twice the number of patients without skull fracture as in those with skull fractures.
Gennarelli found the incidence of fracture to be similar across all severities of brain injuries in 434 patients [11]. There was a slight tendency for the severity of fracture to increase as the severity of brain injuries increased. However, this tendency was not statistically significant. Similar conclusions were drawn by Chapon et al. [1]. Cooper (1982b) states that there is no consistent correlation between simple linear skull fractures and neural injury [10].
Comminuted skull fractures result from severe impacts and are likely to be associated with neural injury. Cooper further states that clinically depressed fractures are significant where bone fragments are depressed to a depth greater than the thickness of the skull. The incidence of depressed skull fracture is 20 per 1,000,000 persons per year, with an associated mortality rate of 11 % related more to the central nervous system (CNS) injury than the depression itself. From 5 % to 7 % have coexisting, intracranial hematoma and 12 % have involvement of an underlying venous sinus [10]. Clinically, basal skull fractures are significant because the dura may be torn adjacent to the fracture site, placing the CNS in contact with the contaminated paranasal sinuses. The patient will be predisposed to meningitis [12].
9.3 Experimental Brain-Injury Models
Mechanical injury to the brain can occur by a variety of mechanisms. General categories include (1) direct contusion of the brain from skull deformation or fracture; (2) brain contusion from movement against rough interior surfaces of the skull; (3) reduced blood flow due to infarction or pressure; (4) indirect (contrecoup) contusion of the brain opposite the side of the impact; (5) tissue stresses produced by motion of the brain hemispheres relative to the skull and each other; and (6) subdural hematoma produced by rupture of bridging vessels between the brain and dura mater. The latter three mechanisms are hypothesized to be involved in both impact and nonimpact (intertial acceleration) head injury [13].
Regardless of the experimental-outcome variable to be studied by the neurotraumatologist, the model chose by the researcher will produce the injury by mimicking one or more of the mechanisms described earlier. Experimental models of brain injury have used different techniques and a variety of species, with the common objective to develop a reproducible model of trauma that exhibits anatomical, physiological, and functional responses similar to those described clinically. Despite these efforts, there is not a single “ideal” experimental injury model; rather the goals and objectives of the research must dictate what is required of the model.
To this end there exists a variety of models. However, there is no entirely satisfactory experimental model that succeeds in producing the complete spectrum of brain injury seen clinically and yet is sufficiently well controlled and quantifiable to be a useful model for experimental studies. Experimental injury models that can be characterized biomechanically are required for physical and analytical modeling of tissue deformation. These modeling efforts will be useful for correlation of experimental results and injury response with human-injury initiation and response. Assuming that neural and vascular tissues are injured by induced local stresses and strains, the technique used to load or deform the neural tissue at the contact surface is unimportant if the pattern of injury seen is comparable to that observed in clinical brain injury. One can argue that since the structural changes, such as contusion, hemorrhage, or axonal injury, are distributed in patterns similar to those observed clinically, the local mechanics of the injury at a tissue level are comparable even if the gross-level input is different [14, 15].
This section will review specific experimental models of brain injury developed to study biomechanical and physiological mechanisms of clinical CNS trauma. The experimental brain injury models will be divided into three general categories: (1) head impact; (2) head acceleration; and (3) direct brain deformation. Examples will be reviewed and discussed for each model category. Finally, the use, value, and appropriate validation of physical and analytical models will be discussed in relation to development of predictive criteria for functional neural injury in humans. The section focuses on experimental models of nonpenetrating mechanical brain injury and will discuss the appropriateness and applications of those models for investigation of injury biomechanics, pathophysiology, and histopathology.
9.3.1 Method Development
The development and evolution of any experimental model of traumatic neural injury depends in part on the investigator’s objectives. Differing scientific objectives and goals may result in different, but equally appropriate, models. A goal of injury prevention through improved understanding of injury biomechanics poses somewhat different constraints and requirements for the design of the model than, for example, testing efficacy of pharmacologic interventions on specific physiologic aspects of the injury response. However, all models must mimic to some extent the mechanical mechanisms of brain trauma observed in real world trauma to assure comparability of mechanisms. Despite differing experimental objectives, there are certain requirements that are common to any successful experimental model for traumatic brain injury: (1) The mechanical input used to produce the injury must be controlled, reproducible and quantifiable; (2) The resultant injury must be reproducible and quantifiable and produce functional deficit and neuropathology that emulate human brain injury; (3) The injury outcome, whether specified in anatomical, physiological, or behavioral terms, must cover a range of injury severities; (4) The level of mechanical input used to produce the injury should be predictive of the outcome severity.
The primary objective for any experimental model of brain injury is to create specific pathophysiologic outcomes in a reproducible manner. The importance of reproducible, intermediate-severity injury levels should be emphasized, because valuable data are likely to be found at transition- or threshold-level regions. Whether one is evaluating the relationship of injury biomechanics to outcome severity or the efficacy of a particular treatment regimen, the range of outcome modification due to altered mechanical input or treatment is likely to be greater at the intermediate-severity level than at either supramaximal or trivial injury-severity levels.
Although the mechanical input to the brain mimics, at least in part, the biomechanics of human trauma, some simplifications are helpful and indeed necessary to enable interpretation of mechanisms of injury at a tissue level. For example, direct brain deformation reproduces only some of the dynamics of closed head-impact trauma, but can be characterized biomechanically. Provided that the injury technique is designed to be relevant to hypothesized biomechanics and pathophysiology or clinical injury, the critical factor becomes the production of functional and anatomic sequelae comparable to those observed in human neurologic injury.
A simplified model, once characterized biomechanically and physiologically, can be combined with analytic and physical models of the neural, vascular, and skeletal structures to define injury-tolerance criteria at the tissue level. A reproducible, well-defined mechanical input simplifies to a degree the interpretation of the pathophysiologic, biomechanical, and biochemical mechanisms of the resultant injury.
9.4 Models of Brain Injury
9.4.1 Head-Impact Models
Nonpenetrating head-impact models can be grouped according to whether the resulting head motion is constrained to a single plane or whether the head is allowed to move freely in an unconstrained manner. These two types of head impact models were first studied in primates and later in non-primate species. Controlled nonpenetrating head impact was pioneered by Denny-Brown and Russell in primates [16, 17].
In early experiments performed by these researchers, the head motion resulting from the impact to the head was unconstrained. Their experiments laid the groundwork for the use of controlled impact to the unrestrained head as a common experimental technique because it was observed that when the head was fixed, cerebral concussion was less likely to result. The neurological and physiological indicators of injury produced by direct impact to the head were evaluated in fully anesthetized animals. Since a surgical plane of anesthesia is required to assure absence of discomfort to the animal during the experimental impact procedures, immediate assessment of the injury severity is limited to brainstem reflexes and systemic physiologic changes.
Gurdjian and associates used an impact piston with a 1-kg mass to strike the head of anesthetized primates at a predetermined location on the skull. Physiologic parameters, impact force, and head acceleration were monitored in an effort to determine thresholds for concussion and coma, coup and contrecoup contusions, and relative brain motions [18–20]. Later experiments performed by Ommaya and others used a molded protective skull cap to prevent fracture. Slight variations of this technique have been used to evaluate changes in intracranial pressure, behavior, systemic physiology, cerebral metabolism and histopathology following impact injury to the primate head [21–28].
Head motion in these primate studies was constrained only by the neck, resulting in complex and poorly characterized three-dimensional head movement. Severity was determined by selection of impactor velocity, impactor mass, interface material, and impactor contact area. However, like human brain injury the potential injury mechanisms remained complex. While a wide range of biomechanical responses exhibiting clinical relevance were likely produced, including local skull deformation, development of pressure gradients within the brain tissue, and relative motion between the brain and skull, because of the dynamics of the resultant head movement, it was not technically feasible to quantify or separate the various biomechanical components of the injury event for more detailed analysis of injury mechanisms. Although some studies attempted to evaluate the effects of rotational and translational head acceleration separately, careful biomechanical analysis of the experiments found that impact to the unrestrained head always produced both rotational and translational acceleration.
The various direct head-impact methods are successful at producing either fatality or short-duration unconsciousness but generally suffer from a high degree of variability in the response. This variability may result from a lack of control over the precise conditions of the impact, an absence of control of head dynamic response, and inconsistent impactor-skull interface parameters. As a result, the expected variability due to biological differences is confounded by mechanical variability of the injury event, necessitating a large number of experiments to obtain a representative sample of pathophysiologic response. In addition, the uncontrolled injury biomechanics make analysis of brain-injury biomechanics or testing of therapeutic efficacy difficult at best.
A number of studies have therefore constrained, at least partially, the head motion during and after impact in an effort to increase the reproducibility of injury outcome [29–31]. Full constraint was not obtained, but the head motion was typically confined to a single plane. An analysis of the pathophysiology and relation of impact biomechanics to outcome in these studies indicates that the partial control of head motion does not represent a significant improvement over the gradation and reproducibility of impact to the unconstrained head.
Closed head impact has been applied to nonprimates, including rat and cat, with limited success [32–39]. Similar to early experiments involving primates, biomechanical interpretation of the test results was initially impeded by the unpredictable head movement resulting from the impact. This was due in part to unspecified level of head support or restraint. As a result, biomechanical analysis of head and brain dynamics following head impact was inconsistent.
Head-impact studies performed in cats, using a captive bolt pistol to deliver the impact, have been most extensively developed by Tornheim [36–39]. Oblique impacts delivered to the coronal suture cause a reproducible contusion with associated edema and skull fracture. The captive-bolt head-impact model has been used successfully to test efficacy of anti-edema drugs. Subsequent experiments have used an oblique lateral impact, with the head resting on a collapsible hex-cell support [36, 38]. This model is useful for studies of cerebral contusion and resultant edema, though reliable gradation of impact severity is still needed.
In general, whole head impact has not been successful at producing clinically relevant brain injury in the rat. In most cases, early attempts using direct head impact to the rat resulted in either no identifiable injury or immediate convulsions associated with apnea. This finding highlights a common problem shared by early whole head-impact models in subprimates, particularly the rat. That problem is the inability to produce a graded, reproducible range of injury outcomes by varying the biomechanical parameters of the impact. In some rat impact models high-level input parameters resulted in either fatality or a brief period of unconsciousness, usually measured by absence of the righting reflex [32, 40–43].
Skull fracture commonly occurs at impact-severity levels insufficient to produce prolonged coma in the rat; moreover, the occurrence of skull fracture is generally not well correlated with injury severity. Convulsive activity, a common result of direct head impact in the rat, may affect overall outcome and must be considered in interpretation of cerebral metabolic, neurophysiologic and electroencephalographic changes which follow injury. The histopathological evidence of neural injury is typically restricted to the lower brainstem in contrast to findings in human brain injury. Finally, the injury response curve is extremely steep; only a slight change in impact parameters is needed to produce an injury spectrum from minor to fatal.
Goldman and collaborators have reported results obtained using a newly developed closed head-injury impact model of mild to moderate head injury in the rat [44]. Moderate concussion in this model is characterized by 4–10 min of unconsciousness in the absence of skull fractures or brain contusions. This new experimental brain-injury model utilizes a pendulum to deliver a controlled impact to the intact skull. The skull and maxilla rest on an energy absorbing rubber mat to reduce the likelihood of a skull fracture. The pendulum impact surface incorporates a load cell that enables measurement of the amplitude and duration of force produced by the impact. This model reproducibly produces brain swelling accompanied by increases in intracranial pressure lasting more than 3 days post-injury. Significant morphologic changes include edema and neuronal death.
The model developed by Goldman is significant and unique in producing a reproducible range of mild- to moderate-severity injury based on pendulum stroke, impact load, and animal body weight, in the absence of skull fracture [44]. Repeatability of experimental outcome is dependent on brain mass and is affected by skull strength. A key step in the success of this model was development of an impact force/bodyweight nomogram that compensates for differences in skull strength over the body-weight range (330–430 g) so that similar cerebrovascular responses are achieved in animals at the extremes of body weight. Well-controlled animal husbandry conditions are critical for the reproducibility achieved by these investigators. Goldman reported variances in estimates of regional cerebral permeability that were well within the range encountered in other studies [45]. Physiologic and morphologic changes parallel many aspects of human head trauma, particularly elevation of intracranial pressure. These observations suggest this may be a useful and relatively inexpensive tool for investigating the mechanisms and therapeutics of brain trauma, and especially edema and elevated intracranial pressure.
9.4.2 Head Acceleration Models
Various head acceleration models have been developed, some of which tightly control the motions of the head and others of which allow free head motion. Free head acceleration is attained through an abrupt deceleration of a moving frame to which the rest of the body is firmly affixed. This produces a whiplash head motion and can result in concussion [26, 28]. These studies have been performed in large nonhuman primates since the anatomic relationships between brain, brainstem, and spinal cord are most similar to man, as is the ratio of brain mass to head mass.
In any whole head acceleration model it is the geometry and mass distribution that will determine the brain dynamics and resultant injury. A significant difference between the head-impact and head-acceleration models is the relative absence of skull fracture in the acceleration models; however, in real situations the range of induced accelerations that produce injury experimentally is only achieved in man when head impact occurs.
Since unconstrained head dynamics make analysis of the injury biomechanics difficult and contribute to outcome variability, the majority of studies using head acceleration techniques have employed some type of helmet and linkage to control the head motions [6, 46]. Such a system controls the path direction, path length, and duration of acceleration so that the head motion is comparable between experiments with a comparable input. In addition, controlling the path of head movement during acceleration reduces the incidence of skull fracture and local skull deformation which are common occurrences in impact techniques.
The most extensive series of experiments studying acceleration induced brain injury was initiated by Ommaya and Gennarelli and concluded by Gennarelli and Thibault. The investigators were able to produce graded anatomic and functional injury severities, including prolonged traumatic unconsciousness (>30 min) in nonhuman primates [6]. This series of experiments built upon earlier head-acceleration studies that had indicated that loss of consciousness was more readily produced by high levels of angular acceleration than high levels of translational acceleration [46, 47, 48]. Consequently, a device and control linkage was designed to allow high levels of angular acceleration to be delivered to the primate head without skull fracture [6].
The technique used a pneumatic thrust column, helmet, and pivoting linkage to apply 60″ of angular rotation within 11–22 ms, attaining peak angular accelerations of 1–2 × 105 rad/s2. The acceleration pulse was biphasic, with a relatively long, ramp-like acceleration phase followed by an abrupt deceleration phase, with injury presumed to occur during the deceleration phase. In addition to prolonged traumatic coma, these animals exhibited diffuse axonal injury in the subcortical white matter, comparable to pathology observed in clinical brain injury [6, 49]. Histopathological evaluation of the brains shows a high degree of comparability to clinical brain injury pathology, substantiating the relevance of the technique [50–52]. The spectrum of injury obtainable using this technique ranges from mild, subconcussive brain injury with only sparse histologic evidence of axonal damage, through prolonged (hours to days) traumatic coma with extensive axonal injury throughout the white matter and brainstem, to immediately fatal injury.
Biomechanical analysis has been partially successful in relating the magnitude of acceleration input to the severity of injury outcome in this model, although interpretation is confounded by the two-phase acceleration-deceleration pulse, which results in nearly comparable acceleration and deceleration force peaks and the potential for neural and vascular injury during either or both pulses. Nevertheless, the need to use nonhuman primates for the technique (because of geometrical considerations) slows the progress of research since it is not possible to perform the large number of experiments necessary to fully use the experimental power of the technique. The injury device is also complex and very costly to duplicate. It requires collaboration of medical scientists and biomechanical engineers for implementation and meaningful data interpretation. Finally, due to the biphasic acceleration-deceleration pulse, conclusions regarding the biomechanics and timing of neural and vascular tissue injury must be drawn cautiously.
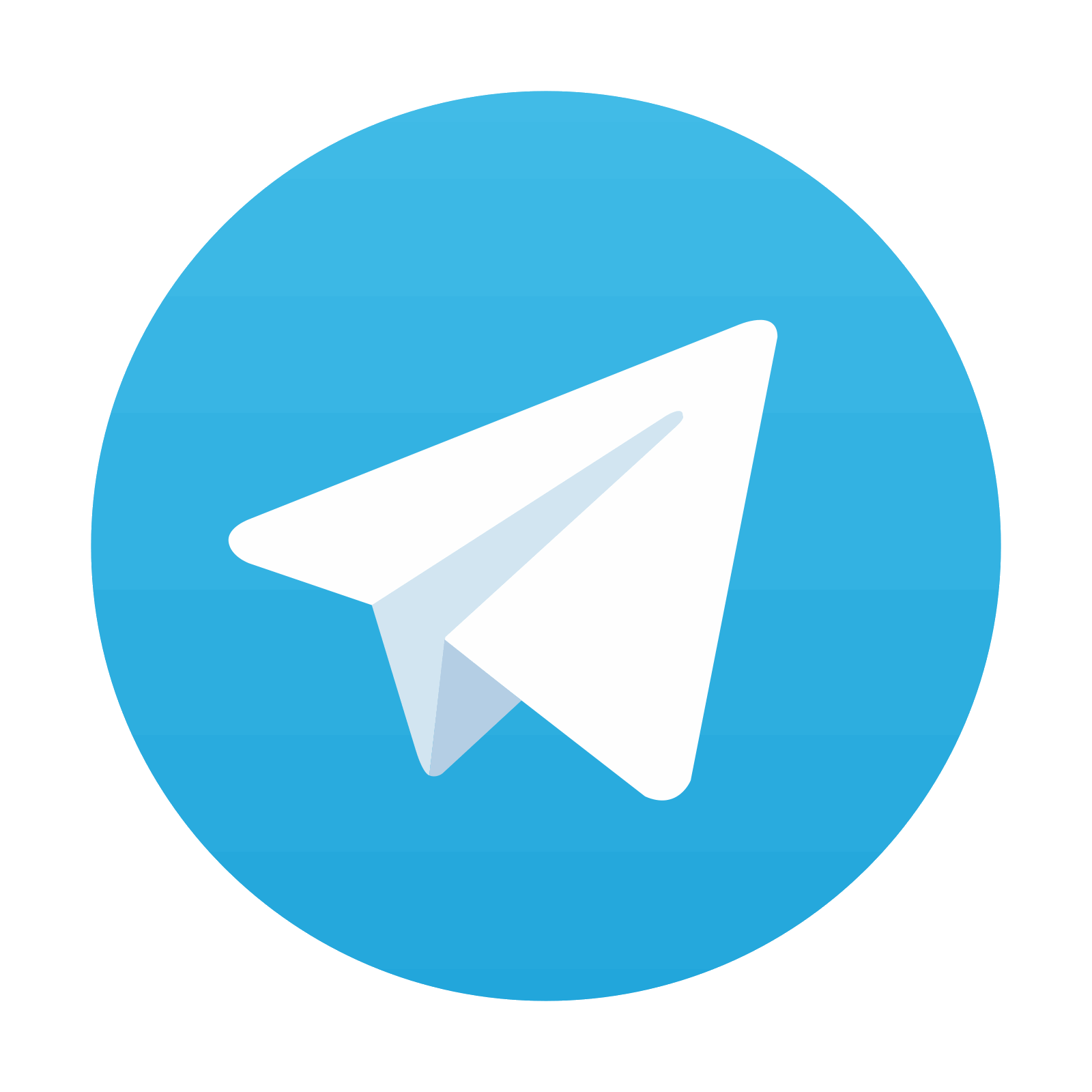
Stay updated, free articles. Join our Telegram channel
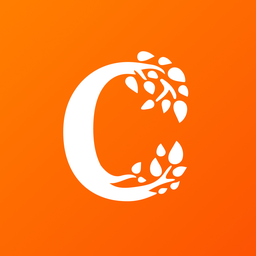
Full access? Get Clinical Tree
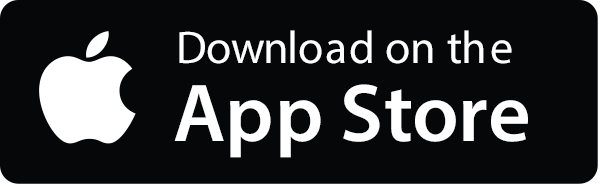
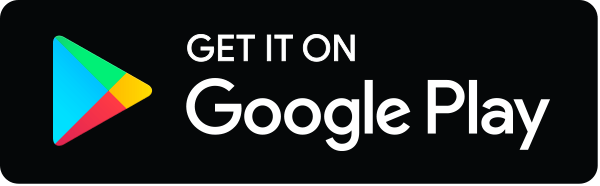