Biology of Bone
Robert J. Majeska, PhD
Oran D. Kennedy, PhD
Mitchell B. Schaffler, PhD
Elise F. Morgan, PhD
Louis C. Gerstenfeld, PhD
Dr. Majeska or an immediate family member has stock or stock options held in Pfizer. None of the following authors or any immediate family member has received anything of value from or has stock or stock options held in a commercial company or institution related directly or indirectly to the subject of this chapter: Dr. Kennedy, Dr. Schaffler, Dr. Morgan, and Dr. Gerstenfeld.
Keywords: age-related fractures; bone biology; histomorphometry; radiological assessments
OVERVIEW OF BONE DEVELOPMENT AND FUNCTION
Bone come in a variety of shapes, sizes, and internal organizational patterns that serve a number of physiological functions with each bone uniquely suited for its functional role.
Functionally, the bony skeleton carries out three primary roles. Structurally, bone is a load-bearing scaffold providing mechanical support to the body and the load-bearing scaffold onto which the musculature is attached. It also provides protection for internal organs of the head, thorax, and abdomen. Metabolically, bone is a reserve source of minerals, particularly calcium and phosphate, for the management of mineral metabolism. Finally, bone plays a major role in organismal homeostasis by housing tissue niches that contain the postnatal stem cell populations supporting hematopoiesis, myelopoiesis, and skeletogeneis. Thus, bone tissues are integral to the regeneration of these organ systems throughout adult life. Thus, while the primary clinical focus of the orthopedic surgeon is on the restoration and maintenance of the skeleton’s mechanical functions in patients, the overall clinical treatment and management of many orthopaedic problems requires an understanding that the etiology of orthopaedic problems is intimately linked to interplay among the entire spectrum of functions carried out by skeletal tissues.1,2,3,4
Bones arise from two embryonic sources. Those of the skull and upper mandible are derived embryonically from the neural crest, by intramembranous bone formation in which cells of embryonic mesenchyme differentiate directly into osteogenic cells. Bones of the vertebrae, ribs, aspects of the pelvis and limbs arise from various elements of the mesoderm via endochondral bone formation, in which an initial cartilage model (anlage) forms within mesenchyme. The cells surrounding the anlage then differentiate into osteoblasts which lay down bone matrix upon it, after which the cartilage is gradually replaced. Details of these developmental processes are available in most anatomy texts and reviewed in Chapter 9.
Endochondral bone formation during skeletal development has several clinically relevant implications for postnatal growth and repair. First, the growth in length of long bones depends primarily on the growth of cartilage in the growth zones; consequently, as long bones are growing in length, regions of cartilage will be evident. Bones grow in length from the first trimester of development in utero until the late adolescence. A second implication is that the majority of bones formed by endochondral processes become sites in which postnatal hematopoietic tissues are housed.
CLINICAL NOTE The development of bones by the endochondral pathway depends critically on the control of cartilage differentiation and growth throughout the developmental process. As a result, many orthopaedic pathologies characterized by skeletal malformations arise from genetic or developmental deficiencies in cartilage rather than bone per se. These include instances of defective skeletal patterning (eg, polydactyly) due to altered expression of transcription factors controlling chondrocyte differentiation and various forms of dwarfism due to impaired function of chondrocytes in growth zones. It is also important to recognize that failures in these developmental processes are causal to many of the problems that impede surgical repair, fracture healing, and surgical treatments. A complete summary of the nosology of known congenital mutations effecting skeletal tissues has been cataloged.5
HIERARCHICAL LEVELS OF BONE STRUCTURE
In the next section, bone structure will be reviewed beginning at the anatomical level, proceeding through tissue-level organization to its underlying molecular composition.
Anatomically, the skeleton is divided into axial elements consisting in the adult of 80 bones including the skull, the vertebral column, sacrum, coccyx, and the thoracic cage. The appendicular skeleton is composed of 126 bones that include all bones of the upper and lower limbs, plus the elements of the pelvis that attach each limb to the axial skeleton. Bones may also be categorized roughly as either long or flat.1,2,3,4 As noted, long bones such as the humerus, femur, and tibia generally form by the endochondral process, whereas flat bones, like those of the cranium, arise via intramembranous ossification. All bones contain a dense outer layer of compact or cortical bone that surrounds a network of thin trabeculae (cancellous bone), with the intratrabecular space filled with marrow. Like size and shape, the amounts and distributions of cortical bone, cancellous bone, and marrow differ greatly among individual bones and influence how they develop, age, and are prone to fracture, as will be discussed below.
The femur illustrates most of the macrostructural features of a prototypical long bone. It roughly resembles a cylinder with fluted ends (Figure 1) with a middle region (diaphysis) flanked by the metaphyses and the epiphyses. The diaphysis consists of a thick shell of compact cortical bone enclosing a marrow cavity with little or no cancellous bone. In the metaphyses and epiphyses, the cortices are thinner and surround a more extensive network of cancellous bone. Marrow occupies the internal spaces of the bone. As noted previously, the epiphyseal plate, or physis, separates the epiphysis from the metaphysis in growing bones, but cartilage disappears from the physeal region after growth ceases.6,7,8
Both external and internal (marrow) surfaces of bone are covered by some form of nonmineralized connective tissue. Externally, the ends of bones that form joint surfaces are covered by articular cartilage, whereas the remainder of bone is covered by the periosteum, a somewhat elastic sleeve of dense fibrous connective tissue. The periosteum includes a layer of cells near the bone surface (the cambium layer) that contains both skeletogenic stem cells and osteogenic progenitors. These cells are capable of forming bone and cartilage cells that contribute to growth, response to injury and homeostatic maintenance of bones. Internally, surfaces of both cortical and cancellous bone are covered by the bone marrow stroma, a fibrous connective tissue more loosely organized than the periosteum. Cells of the marrow stroma comprise a heterogeneous population that plays several roles in maintaining both the hematopoietic system and connective tissue elements of the bone. Hematopoietic stem cells that give rise to all blood cell types reside physically in or around the stroma, whereas connective tissue cells within the stroma produce growth factors needed to sustain the hematopoietic stem cells and to support their differentiation. In addition, bone marrow stroma contains a population of nonhematopoietic adult stem cells (marrow stromal stem cells or MSCs) capable of differentiating into bone, cartilage, tendon, and adipose tissues, among others. Like all tissues, bone is supplied by blood vessels and nerves.8,9 These are more extensively distributed throughout the periosteum and the bone marrow than in bone itself. Vessels and nerves that supply the marrow pass through cortical bone only at limited entry points (nutrient foramina). Smaller vessels and nerves are distributed throughout cortical bone, passing through channels in the tissue termed haversian canals and Volkmann canals (discussed below). The extent of intracortical vasculature varies considerably among individual bones and certainly among species. In bones that lack haversian systems, primary vascular canals are still present, running in both longitudinal and transverse directions. Figure 2 presents a schematic summary of this haversian cortical bone structure.
The blood vessels in bone derive from both periosteum and marrow, which supply the outer one-third and the inner two-thirds, respectively. Marrow vasculature also services trabecular bone, which itself does not contain vessels. There is a second so-called circulatory system in bone—the extravascular movement of fluids and solutes through the pericellular space between osteocytes and their processes and their bony lacunar and canalicular walls; fluid in this space moves under convective flow driven by blood pressure and by mechanical
loading. Aspects of fluid flow and tissue mechanoregulation will be further discussed below. Whether bone has lymphatic drainage remains unresolved.9
loading. Aspects of fluid flow and tissue mechanoregulation will be further discussed below. Whether bone has lymphatic drainage remains unresolved.9
TISSUE-LEVEL ORGANIZATION (GROSS AND MICROSCOPIC)
Tissue-level (or intermediate) organization considers the way cells and extracellular matrix (ECM) are arranged within bone tissue at the microscopic length scale; its description is historically based largely on microscopy and histologic staining methods.1,7,10 Cortical and cancellous bone can be characterized as either woven or lamellar. Woven bone is a poorly organized tissue in which neither the bone cells nor the fibers in their surrounding matrix exhibit any organized arrangement. Most notably, the collagen fibers in woven bone matrix are randomly oriented. Woven bone is produced during periods of rapid bone formation; for example, during development, in fracture callus, and in primary bone tumors (osteosarcomas). In nonpathologic circumstances, woven bone is a temporary or provisional tissue that is removed and replaced by more highly organized lamellar bone, which comprises effectively all adult cortical and cancellous bone tissue. Lamellar bone consists of tissue layers (lamellae) approximately 3 to 5 µm thick, in which the collagen fibers run mainly in the same direction. The orientation of fibers in adjacent lamellae, however, differs by as much as 90°, giving lamellar bone a “cross-ply” or plywood-like appearance that is particularly evident under polarized light microscopy. Biological mechanisms by which collagen fibers become arranged in successive lamellae are not understood, but have an overall significance to the mechanical strength of the tissue.
In cortical bone, lamellae are generally organized in one of several ways. (1) Circumferential lamellae extend continuously around some or a bone’s entire circumference. The most prominent example is the deposition of effectively complete circumferential sheets on the periosteal surface of bone during radial growth of diaphyses (“outer circumferential lamellae”). (2) Circumferentially oriented lamellae are added to parts of
the endosteal surface as well (“inner circumferential lamellae”), although these often do not extend the full extent of the inner surface of the bone. (3) Osteonal or haversian lamellae are concentric rings of tissue that lie inside an osteon or haversian system—a cylindrical region that surrounds a vascular channel within bone. (4) Interstitial lamellae are remnants of lamellae that lie between haversian systems and can be composed of fragments of circumferential lamellar bone from earlier times in a bone’s growth, or of fragments of preexisting haversian systems. The vascular canals that are roughly aligned with the long axis of the bone are termed haversian canals, whereas those that run transversely are called Volkmann canals. The borders of each osteon are delineated by a thin layer of distinctive matrix that is termed a cement line. The cement line is a bonding layer formed after the old matrix has been removed during remodeling and before new bone matrix is deposited.
the endosteal surface as well (“inner circumferential lamellae”), although these often do not extend the full extent of the inner surface of the bone. (3) Osteonal or haversian lamellae are concentric rings of tissue that lie inside an osteon or haversian system—a cylindrical region that surrounds a vascular channel within bone. (4) Interstitial lamellae are remnants of lamellae that lie between haversian systems and can be composed of fragments of circumferential lamellar bone from earlier times in a bone’s growth, or of fragments of preexisting haversian systems. The vascular canals that are roughly aligned with the long axis of the bone are termed haversian canals, whereas those that run transversely are called Volkmann canals. The borders of each osteon are delineated by a thin layer of distinctive matrix that is termed a cement line. The cement line is a bonding layer formed after the old matrix has been removed during remodeling and before new bone matrix is deposited.
CELLULAR LEVEL ORGANIZATION OF THE BONE AND THE CELLULAR ASPECTS BONE HOMEOSTASIS
All bone contains four distinct cell types: osteoblasts, osteoclasts, osteocytes, and bone lining cells. These cells are involved in the maintenance and metabolic functions of bone and carry out primarily either anabolic or catabolic functions. Osteocytes and osteoblasts synthesize and secrete the organic constituents of bone matrix and regulate its mineralization; osteoclasts degrade (resorb) bone, simultaneously breaking down mineral and matrix. Osteocytes and lining cells are bone’s long-term residents that carry out day-to-day maintenance of the tissue; they are found, respectively, within the mineralized matrix and on both cortical and trabecular surfaces not being formed or resorbed.
LINEAGES
Osteoblasts, lining cells, and osteocytes are part of a common mesenchymal cell lineage, differentiating from skeletal stem cells and progenitors that reside in specific tissue niches within the bone marrow and within the periosteum adjacent to bone proper. Osteocytes and lining cells are, in fact, late—and alternative—stages in the phenotypic maturation of osteoblasts. As osteoblasts lay down new matrix, some of them become buried within it and become osteocytes (some other osteoblasts die by apoptosis). When formation ceases, the last osteoblasts flatten out to cover the new matrix and become lining cells. Osteoclasts, by contrast, arise from the hematopoietic lineage,1,2,7,10,11,12,13,14,15,16 specifically monocytes which are found in the marrow and recruited to potential resorption sites where they differentiate into multinucleated osteoclasts that resorb bone until the appropriate amount has been removed. Both osteoblastic and osteoclastic lineages have been extensively studied so that phenotypic markers—and in particular, critical transcription factors responsible for their distinctive gene expression patterns—have been identified for most stages of differentiation from stem cells onward (Figure 3, A and B). The osteoclast lineage appears to include few if any branches or reversals. On the other hand, the skeletogenic stem cells giving rise to osteogenic cells also give rise to cartilage cells, fibroblasts, and adipocytes. Moreover, cells within the osteoblastic lineage appear to have considerable plasticity and appear to be capable of phenotypic switching or reversal under differing circumstances, both in vivo and in vitro. Lining cells can be activated rapidly to become osteoblasts in vivo17; in addition, a substantial proportion of cells from
adult bone exhibiting an osteoblastic phenotype in culture18 were almost certainly osteocytes in vivo. Finally, osteoblast progenitors have the option to differentiate instead into adipocytes, and the propensity for each m100y change with age and/or may contribute to diminished bone-forming capability in aging and certain pathological conditions.19
adult bone exhibiting an osteoblastic phenotype in culture18 were almost certainly osteocytes in vivo. Finally, osteoblast progenitors have the option to differentiate instead into adipocytes, and the propensity for each m100y change with age and/or may contribute to diminished bone-forming capability in aging and certain pathological conditions.19
FUNCTIONS
The function of osteoblasts, as stated previously, is to produce the organic matrix of bone and help regulate its mineralization. Details of these activities will be discussed later in the chapter. However, it should be noted that osteoblasts are postmitotic; they do not divide. New osteoblasts are supplied from ranks of osteoblast progenitors lying “behind” the osteoblasts, away from the bone surface.
Osteocytes are the most abundant cells in bone and carry out much of the signaling throughout the tissue. Osteocytes occupy roughly ellipsoidal 300 to 500 µm3 spaces (lacunae) and connect with each other through extensive networks of dendritic processes. These processes are located in canaliculi approximately 0.3 µm in diameter and are joined by gap junctions where they connect at process termini. In lamellar bone, the lacunae are regularly arranged and consistent with the organization of the lamellae; in woven bone, lacunae are arranged randomly. The lacunar-canalicular system (LCS), the pericellular space surrounding osteocytes and their processes, provides the major route through which fluids, nutrients, signaling molecules, wastes, and other molecules move through bone. It is hypothesized that both the fluid movement and small amounts of tissue deformation that is applied on the dendritic processes and their interconnection is how osteocytes sense mechanical loading.11,20
In addition to acting as mechanosensors, osteocytes have been implicated as effector cells regulating several major homeostatic functions of bone. Osteocytes upregulate the pro-osteoclastogenic cytokine RANKL in response to apoptosis induced by remodeling stimuli that include microdamage, estrogen deprivation, and mechanical unloading. Osteocytes also produce sclerostin, the Wnt antagonist that suppresses osteoblast activity. In addition, osteocytes can regulate local remodeling of the LCS in association with mineral exchange; this is especially evident in lactation and can lead to rapid, though temporary, changes in local material properties of bone. Finally, osteocytes exert systemic actions on the kidney via FGF-23 and PHEX, which is mutated in x-linked hyperphosphatemia.21
Osteoclasts—the cells responsible for the wholesale removal of bone at a defined site—are notably polarized cells. Osteoclasts attach to bone surfaces with specialized structures forming a resorption space that encloses a “ruffled border” of active plasma membrane. Protons generated by carbonic anhydrase within the cytoplasm are pumped into the resorption space via transport molecules in the ruffled border. Intracellular pH and ionic balance are maintained by additional ion transport systems located in the plasma membrane outside the ruffled border. The secreted protons, which render the resorption space acidic, begin to dissolve the apatite mineral salts and disrupt intramolecular and intermolecular interactions of organic constituents. Osteoclasts also secrete lysosomal enzymes including tartrate-resistant acid phosphatase (TRAP, an enzyme that has served as a convenient, histochemical marker to specifically identify osteoclasts) and proteases (most notably cathepsins B, L, and K).12,13,14,15
As the effector cells responsible for bone removal, osteoclasts have long been a focus of interest in understanding the mechanisms of pathologic bone loss and a target for therapeutics. The role of osteoclasts in mineral metabolism and in the homeostatic maintenance of skeletal structure throughout life is extensively reviewed in Chapter 24. Of particular importance in this regard are the pathways and mechanisms of communication between the osteoblastic and osteoclastic lineages. These include induction of osteoclast differentiation by osteocytes and osteoblasts by the obligate factors RANKL (receptor activator of NF-kappa-B ligand) and M-CSF (macrophage colony-stimulating factor) and the coupling between bone resorption and formation. Advances in understanding these processes have been exploited in the development of antiresorptive therapies to treat osteoporosis (also reviewed in Chapter 24).
MOLECULAR LEVEL OF BONE COMPOSITION AND ITS EXTRACELLULAR MATRIX
The molecular composition of bone tissue is described in Figure 4. It is broadly made up of organic components comprising ˜30% of its mass. The organic component itself is made up of extracellular proteins comprising 98% of its mass and 2% of its mass made up of the cells found embedded within bone or on its surfaces. The other ˜70% of its mass is accounted for by mineral, of which 95% is made up of a carbonated hydroxyapatite and the remainder being other trace minerals.
ORGANIC ELEMENTS OF BONE/COLLAGEN
At the molecular level bone extracellular matrix (ECM) proteins are composed of ˜90% type 1 collagen and 10% noncollagenous proteins The fibers of collagen and noncollagenous proteins, in conjunction with the apatitic mineral crystals precipitated within and around the fibers, form a unique composite material. Broadly speaking, the fibrous matrix governs the tissue’s tensile properties and fracture toughness whereas the mineral phase confers rigidity and compressive strength; however, this is clearly an oversimplification. The organic constituents of bone matrix can have substantial effects on bone’s mechanical properties, such as resistance to fracture, and on its physiologic activities in mineral metabolism,22,23,24,25 as well as playing a wide variety of physiological functions summarized in bottom half of Figure 4. As a major repository of calcium
and phosphate in the body, bone ECM contributes to systemic mineral metabolism.1,3,4,22
and phosphate in the body, bone ECM contributes to systemic mineral metabolism.1,3,4,22
As stated above, type I collagen is the major collagen isotype in bone. Other collagen types found there belong to cartilage remnants from endochondral ossification (mainly types II and X), fibrous tissues of periosteum and marrow (type III), or blood vessels (type IV). Small amounts of other collagen types, including types III, V, and XI, have been identified in bone ECM, but under restricted circumstances and in association with type I fibers.25 As the major organic component of bone, the biosynthesis and molecular structural assembly of collagen type I collagen is integral to overall structural nature of the composite material of bone. The structural unit of type I collagen is termed tropocollagen, a trimer composed of three polypeptide chains. Two are α1 (I) chains, encoded by the col1a1 gene, whereas the third, α2 (I), is encoded by the col1a2 gene. The three chains form a distinctive unit, in which the polypeptides wrap around each other for most of their length, forming a tight triple helical braid. This triple helical structure, which is not the same as the α-helix that is formed by a single polypeptide chain, is the defining structural feature of all collagens. The collagen triple helix forms because both the α1 and α2 chains contain repeated sequences of the amino acid sequence (-gly-X-Y-) where gly represents glycine, X is proline, and Y is frequently hydroxyproline. Both proline and hydroxyproline are imino acids; that is, the nitrogen that combines with adjacent amino acid to form the polypeptide backbone exists as part of a five-membered ring and is not able to rotate freely. This constrained structure imparts a tight kink in the polypeptide chain at each proline or hydroxyproline residue. The positioning of glycine, which lacks a side chain, at every third position along this repeating tripeptide sequence (and adjacent to a proline) is essential to prevent steric hindrance that would otherwise impair wrapping of the helical braid. Fibril formation is a self-assembly process, at physiologic pH, temperature, and ionic strength; monomeric tropocollagen in solution in vitro will spontaneously aggregate in a regular fashion to form fibrils. The spontaneous nature of fibril formation suggests that most or all tropocollagen will exist as part of a fibril and, moreover, that mechanisms must exist in vivo to ensure that the fibrils are of the appropriate dimensions and that fibril formation only occurs under the correct circumstances.
The triple helical region of tropocollagen comprises the central region of the molecule; the remainder includes nonhelical N- and C-telopeptides flanking the helical region on each side, as well as N- and C-terminal propeptides that are cleaved during posttranslational processing. Because the long helical region makes up most of the sequence of mature collagen molecules, each tropocollagen unit resembles a fairly stiff rod with a consistent length of approximately 300 nm. In the fibrils, the rod-like tropocollagen monomers are aligned in the same direction, but with the ends of the monomers offset by approximately 25% of their length—a quarter-stagger configuration relative to the collagen monomers immediately laterally. Thus, the tips of each tropocollagen molecule do not contact each other; rather, there are gaps (holes) between successive tropocollagen units in a line. This regular arrangement of monomers and gaps accounts for the characteristic banding patterns observed in electron microscopic images of collagen fibrils. Because of this arrangement, the addition of each new tropocollagen monomer to a growing fibril via this staggered, side-to-side interaction of monomers results in changes to the width as well as the length of the fibril. The fibrils formed by collagen can be very long, but are roughly circular in cross section and have a limited distribution of diameters that is tissue type dependent. Collagen fibril diameter appears to depend on many factors, including the association of other collagen types (minor collagens such as type V), proteoglycans, or noncollagenous proteins with the fibrils. The presence of holes within the fibril structure of collagen also has implications for the distribution of noncollagenous elements, especially mineral crystals, in bone ECM. The hole zones between the ends of tropocollagen molecules define a network of spaces that extends throughout the three-dimensional extent of the collagen fibrils and is also continuous with interfibrillar space. The basic biochemistry and biology of collagens have been extensively reviewed.22,23,24,25 Consequently, tissue fluid and its soluble components, including noncollagenous proteins and inorganic ions, can permeate into as well as between collagen fibrils. Because of this accessibility, bone mineral crystals are
closely integrated into the fibrils of bone ECM.26 The mechanisms of collagen triple helix assembly, posttranslational modifications, fibril assembly and packing, and interactions with mineral are schematically depicted in Figures 5 and 6.
closely integrated into the fibrils of bone ECM.26 The mechanisms of collagen triple helix assembly, posttranslational modifications, fibril assembly and packing, and interactions with mineral are schematically depicted in Figures 5 and 6.
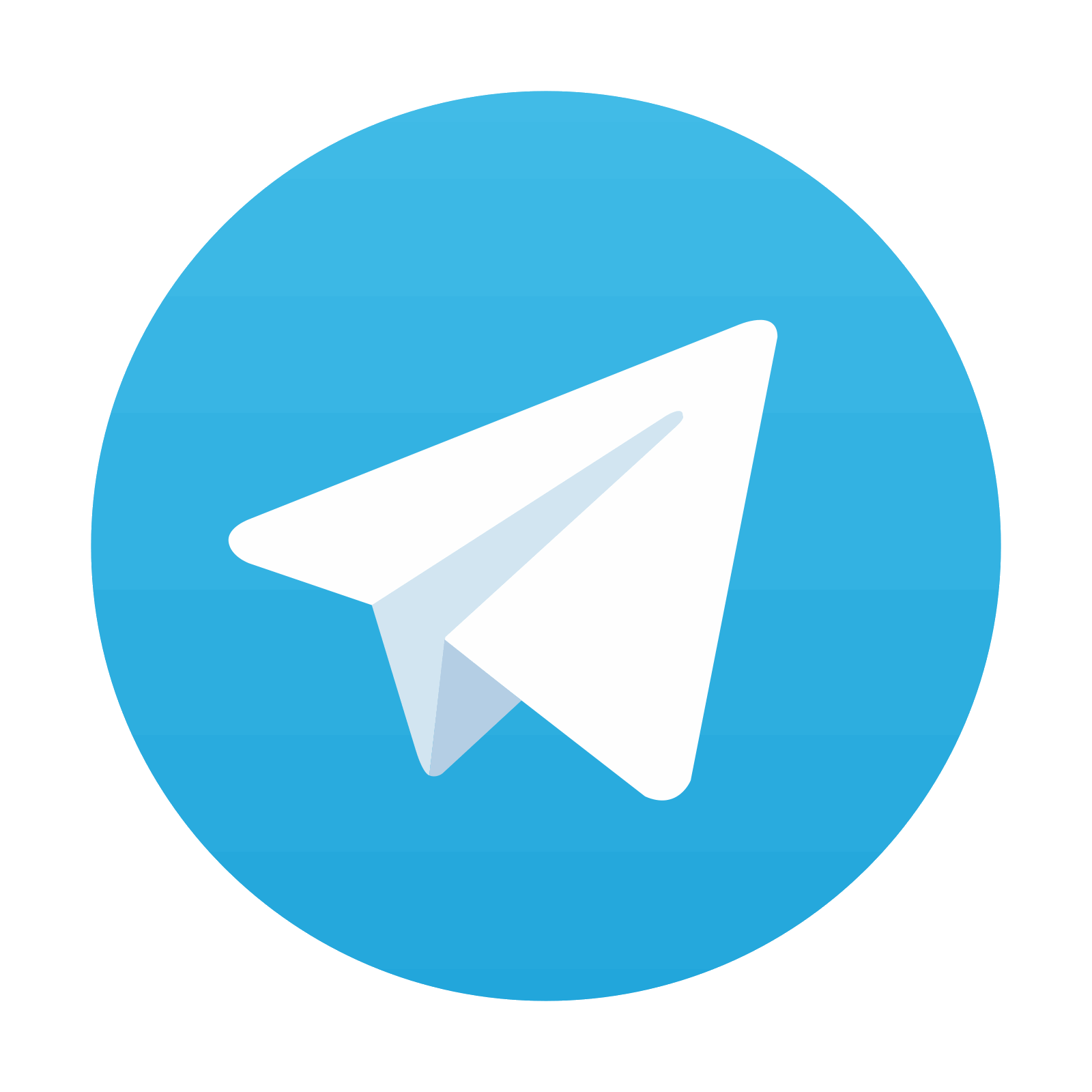
Stay updated, free articles. Join our Telegram channel
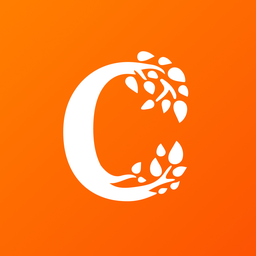
Full access? Get Clinical Tree
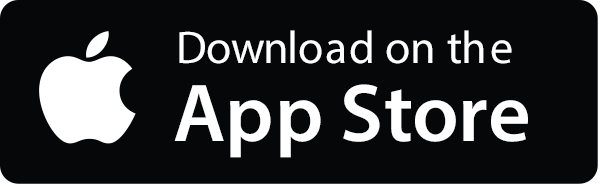
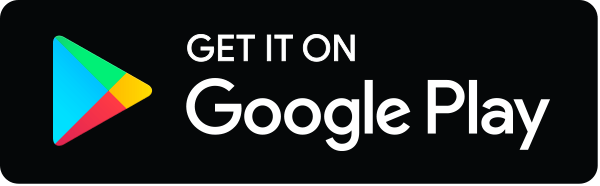