Abstract
The ligaments of the knee function as static stabilizers of a dynamic joint. The anterior cruciate ligament (ACL) is commonly injured during sports; subsequently, ACL reconstruction is one of the most prevalent procedures in the field of orthopedic surgery. Therefore, knowing basic knee anatomy, ACL graft options, and the biologic healing process is critical for understanding how to treat this injury. Current research is focused on using biologic agents to enhance orthopedic surgeries. Innovative technology including platelet-rich plasma, stem cells, and biologic scaffolding shows promise for improved outcomes after ACL reconstruction.
Keywords
Biologics, Bone marrow, Knee, Ligament, PRP, Scaffold
Knee Anatomy and Biomechanics
The knee joint is the largest and most complex joint in the human body. The ligaments of the knee, which provide structural stability to the joint, are particularly vulnerable to injury. The knee consists of two bony articulations, one between the femur and the tibia creating the tibiofemoral joint, and one between the patella and femur creating the patellofemoral joint. The primary function of the knee ligaments is to control normal kinematics, to stabilize the knee, and to prevent abnormal displacement and rotation that may damage articular surfaces. Ligaments of the knee function as the most important static stabilizers and are composed of collagen, elastic, and reticular fibers. Parallel collagen-fiber bundles enable ligaments to bare axially directed tensile loads.
The ligament-to-bone interface is divided into four zones: (1) the ligament substance, (2) fibrocartilage matrix, (3) mineralized fibrocartilage, and (4) bone. This composition is designed to reduce the chance of failure by distributing stress at the bone ligament interface in a gradual fashion.
Fig. 11.1 represents the load-elongation curve for tensile failure of the anterior cruciate ligament (ACL) illustrated by Cabaud. The figure illustrates the ability of the ligament to resist tensile loading. As more load is applied to the knee, ligament fibers straighten, and the ligament elongates. The slope of the curve represents the stiffness of the ligament, whereas the area under the curve represents energy absorbed by the ligament. The initial portion of the graph labeled “clinical test” represents the amount of stiffness that can be elicited during a clinical examination of the knee. The second portion of the graph labeled “physiologic load” is a near linear relationship between load and joint displacement and is characterized by the elastic deformation of the ligament. The yield point represents the point of load beyond which injury to the ligament occurs, followed by a steep drop in load which represents failure of the ligament.

Cruciate Ligaments
The cruciate ligaments consist of a highly organized collagen matrix that accounts for approximately three-fourth of their dry weight. The majority of the collagen is of type I (90%), and the remainder is type III (10%). The cruciate ligaments are named for their attachments on the tibia and are essential for knee joint function. They act to stabilize the knee joint and prevent anteroposterior displacement of the tibia on the femur. The presence of numerous sensory endings also implies a proprioceptive function. They receive the majority of their blood supply from the middle geniculate artery.
The ACL originates from the medial surface of the posterior lateral femoral condyle and courses anteriorly, distally, and medially to the tibial attachment, which is a wide depressed area anteriolateral to the medial tibial spine in the intercondylar fossa. The average length of the ligament is 38 mm, and the average width is 11 mm. The ACL is the primary static stabilizer against anterior tibial translation. Biomechanical testing has shown that the ACL provides an average anterior translation restraint of 87.2% of the applied load at 30 degrees flexion and 85.1% at 90 degrees flexion. The ACL consists of two bundles, the anteromedial bundle which is tight during knee flexion, and the posterolateral bundle which is tight during knee extension. The ACL also plays a lesser role in resisting internal rotation. The maximum tensile strength of the ACL is approximately 1725+/− 270N.
The posterior cruciate ligament (PCL) originates from the lateral surface of the posterior medial femoral condyle in the intercondylar notch, courses distally, and attaches to the tibia in a depression, posterior to the intraarticular surface of the tibia and extends distally 1 cm. The PCL has an average length of 38 mm and an average width of 13 mm. The PCL is considered the primary stabilizer of the knee because it is located close to the central axis of the rotation of the joint and is almost twice as strong as the ACL. The PCL has been shown to provide approximately 95% of the total restraint to posterior translation of the tibia on the femur. It consists of an anterolateral and posteromedial bundle. The anterolateral bundle forms the majority of the PCL and is taut during knee flexion, whereas the posteromedial bundle is smaller and is taut during knee extension. Injuries to the PCL are less common than injuries to the ACL and usually result from hyperextension or an anterior blow to a flexed knee. Of significant degenerative changes that involve the medial compartment, 90% of cases have been associated with chronic PCL injuries.
Collateral Ligaments
The medial collateral ligament (MCL or tibial collateral ligament) connects the medial epicondyle of the femur to the medial tibia and serves to stabilize the knee specifically during valgus knee stress. The MCL consists of superficial and deep portions. The superficial MCL, as described by Brantigan and Voshell, consists of parallel and oblique portions. The anterior parallel fibers arise from the sulcus of the medial epicondyle of the femur and consist of heavy, vertically orientated fibers coursing distally to insert on the medial surface of the tibia. This insertion is on average 4.6 cm inferior to the tibial articular surface and is just posterior to the insertion of the pes anserine. The posterior oblique ligament and deep fibers of the MCL run from the medial epicondyle and blend to form the posteromedial knee joint capsule. The superficial MCL functions as the primary restraint against valgus stress, a restraint to external rotation of the tibia, and a weak restraint to anterior tibial translation in ACL-deficient knees. The parallel fibers of the superficial MCL are under tension from full extension to 90 degrees of flexion but become maximally taut at 45–90 degrees of flexion. The deep MCL extends from the femur to the midportion of the peripheral margin of the meniscus and tibia. Anteriorly, the deep MCL is clearly separated from the superficial MCL by a bursa, but posteriorly the layers blend together. The deep MCL also functions as a weak secondary stabilizer against valgus stress.
The lateral collateral ligament (LCL or fibular collateral ligament) serves as restraint during varus stress of the knee. The LCL originates on the lateral epicondyle of the femur just anterior and distal to the origin of the gastrocnemius. It runs beneath the lateral retinaculum to insert on the head of the fibula, where it blends with the insertion of the biceps femoris. In biomechanical studies the LCL provides 55% varus restraint at 5 degrees of knee flexion and 69% restraint at 25 degrees of knee flexion. There is decreased varus resistance from the posterolateral capsule as the knee is flexed, leading to an increase in total varus restraint by the LCL.
Other Ligaments
Another important ligament of the knee is the medial patellofemoral ligament (MPFL), which serves to stabilize the patella. The MPFL connects the medial border of the patella to the femur and prevents lateral translation of the patella. Anteriorly, the transverse ligament (or anterior intermeniscal ligament) of the knee connects the anterior edges of the medial and lateral menisci. The transverse ligament prevents anterior translation of both menisci during knee extension. Collectively, the posterolateral corner (PLC) also plays a role in knee stability. The static stabilizers of the PLC include the LCL, the popliteus tendon, and the popliteofibular ligament. The PLC functions primarily to resist varus force, as well as posterolateral rotation of the tibia, especially when the cruciate ligaments are deficient. Cruciate tears often accompany PLC injuries, as both are strong stabilizers of the knee.
Graft Options for ACL Reconstruction in 2018
As ACL reconstruction surgeries have become more frequent, the graft options have also expanded to include traditional autografts and allografts as well as synthetic grafts and xenografts. Autografts involve the use of the patient’s own tissue, including options such as hamstring, patellar, and quadriceps tendons. Allografts are donated tissue from cadavers, which also include hamstring, patellar, and quadriceps tendons in addition to achilles and tibialis anterior/posterior tendons. Synthetic grafts, which may be composed of various materials such as Gore-Tex, polypropylene, and so forth, are a less popular option but are an area of future research in ACL reconstruction. Finally, xenografts are similar structures found in a different species which may be used rather than a human cadaveric donor. In choosing the appropriate graft for a patient, many characteristics are taken into consideration including the patient’s age, level of activity, and comorbidities.
Hamstring Autograft
Hamstring autografts are frequently used by harvesting the patient’s ipsilateral semitendinosus and/or gracilis tendons from their sight of insertion on the tibia. Hamstring tendon grafts are often harvested through the same incision through which the surgeon drills the tibial tunnel for the ACL reconstruction, which decreases donor-site morbidity and improves cosmesis. In a study by Gupta et al., patients treated with a hamstring autograft experienced less postoperative pain for up to 6 hours than those treated with bone patellar tendon bone (BPTB) autografts. However, 6–48 h after operation, patients treated with either hamstring or BPTB autografts showed similar pain on a visual analog scale. Hamstring tendons tend to have less long-term postoperative pain associated with them than other autografts such as BPTB autografts, which have been correlated with kneeling pain. A meta-analysis revealed 17.4% kneeling pain in the patellar tendon group compared with 11.5% kneeling pain in the hamstring group. Numerous studies have compared the strength of various graft types. Hamner et al. demonstrated quadruple hamstring tendon grafts to have greater strength and stiffness than patellar tendon grafts during biomechanical analyses (quadruple hamstring tensile load = 4090 N; stiffness, 776 N/m).
Downsides to the hamstring autograft include graft-harvesting morbidity related to sore hamstrings and subsequent weakened knee flexion. The deficit in knee flexion is thought to be more significant at greater flexion angles. Aune et al. found this knee flexion deficit to be statistically significant at the 6-, 12-, and 24-month follow-up when compared to patellar tendon grafts ( P < .01; mean flexion at 240 degrees/s relative to unaffected side in hamstring group = 80%–90% compared with near 100% in patellar tendon group). Patients who undergo hamstring autograft must often adhere to a longer, more cautious recovery. The reason for this is because the graft lacks the bony component similar to that of the BPTB graft, requiring more time for integration into the native femur and tibia. Rodeo et al. reported that soft tissue–bone grafts such as the hamstring graft incorporate into the subject’s bone within 8–12 weeks, which is about 2–6 weeks longer than grafts containing bone. In addition, Brophy et al. analyzed the Multicenter Orthopaedic Outcome Network (MOON) data from 2002 to 2005 and demonstrated an increased risk of infection postoperatively in hamstring autografts when compared with that in BPTB autografts (odds ratio [OR] = 4.6 [95% confidence interval (CI) = 1.2 to 17.9; P = .026).
Bone Patella Tendon Bone Autograft
BPTB autografts consist of harvesting the patient’s ipsilateral middle third of the patellar tendon with adjacent bone from the patella and tibia. The inclusion of bone plugs in the autograft provides bone-to-bone healing and is thought to have faster incorporation than other soft tissue graft types. For this reason, some argue that the use of BPTB autografts for ACL reconstruction is better suited for young, highly active patients desiring a quick recover to high-intensity sports.
Significant kneeling pain and decreased knee extension due to quadriceps weakness postoperatively are two downsides to the BPTB autograft. Corry et al. showed that at 1-year postoperatively, 55% of patients who received a BPTB autograft experienced kneeling pain compared with 6% of patients who received a hamstring autograft. At 2 years, the number for BPTB autograft patients experiencing kneeling pain dropped to 31% and remained at 6% in hamstring autograft patients. A 21-study meta-analysis revealed 17.4% kneeling pain in the patellar tendon group compared with 11.5% kneeling pain in the hamstring group. Some argue that kneeling pain can be decreased by implementing a more rigorous rehabilitation program. Researchers found less patellofemoral pain with an accelerated rehab, attributing the improvement to early range of motion and quadriceps strengthening. Corry et al. also demonstrated a significant amount of thigh atrophy compared to the contralateral leg with BPTB grafts, indicating a decreased extensor mechanism at 1 year after surgery. This difference, however, was no longer significant at 2-year follow-up. BPTB graft harvesting also introduces the risk of fracture in the proximal tibia and patella. Other complications include damage to the patellar articular cartilage, increased risk of patella tendon rupture, and risk of damage to the saphenous nerve below the patella. Though the BPTB autograft may offer a quicker return to high-intensity activity, pain with kneeling, quadriceps weakness, and harvest morbidity must be considered when using a BPTB autograft.
The choice between the two most popular autografts for ACL reconstruction, BPTB versus hamstrings, remains a debate today. Poehling-Monaghan et al. performed a systematic review of 12 publications investigating ACL graft choice and found no statistically significant differences between the BPTB and hamstring autografts in regard to manual laxity or graft failure rates. Manual laxity was measured via Lachman and pivot-shift tests, and instrumental laxity was measured using a KT-1000 arthrometer. They did find that BPTB grafts were associated with increased kneeling pain as well as an increased frequency of osteoarthritis after 5 years. In terms of clinical outcomes measured by International Knee Documentation Committee (IKDC), Lysolm, and Tegner scores, no significant difference was noted between hamstring and BPTB autografts. In another meta-analysis, Samuelsen et al. found a small increased risk in graft rupture requiring revision in hamstring grafts compared to BPTB grafts (2.84% compared to 2.80% in the BPTB grafts), with a number needed to treat of 235.
Quadriceps Autograft
Although less common, quadriceps autograft has shown to be another viable option for ACL reconstruction, and in recent times, it has been gaining popularity. Slone et al. performed a systematic literature review of 14 studies and found the quadriceps tendon autograft to be a safe, reproducible, and versatile graft in regard to ACL reconstruction. The quadriceps tendon is harvested from the patient’s ipsilateral knee using an anterior approach proximal to the patella. In a study by Lee et al. with patients treated with hamstring autografts versus quadriceps autografts, they were found to have similar knee joint stability and functional outcomes postoperatively. The quadriceps tendon autograft had a better outcome in terms of knee flexion than the hamstring graft. Chen et al. found only mild harvest-site tenderness in a group of 12 patients who received a quadriceps autograft at 18-month follow-up, and Fulkerson et al. reported no quadriceps morbidity in their 28-patient study.
The quadriceps graft can be difficult to harvest as the bone is denser, curved, and in close proximity to the suprapatellar pouch. The lack of long-term quadriceps tendon studies has made it a less popular choice than other autograft options, though it is gaining popularity in both research and usage. It remains clear that more research including direct comparison studies between BPTB, hamstring, and quadriceps autograft tendons for ACL reconstruction is needed to provide a better understanding of outcomes.
Allograft
An alternative to using a patient’s own tissue is allograft from cadaveric tissue. Typical allografts include patellar, hamstring, quadriceps, achilles, or tibialis anterior/posterior tendons from a cadaveric donor. The benefits of allograft for ACL reconstruction include decreased morbidity and postoperative pain because the tissue is not harvested from the patient. The lack of donor-site morbidity may also increase the esthetic outcome for the patient, as there are no additional incisions made for graft harvesting. Allografts are associated with shorter surgery time, smaller incisions, less postoperative pain, and hence a quicker return to activities of daily living. Shino et al. reported good to excellent results in 94% of patients receiving an Achilles or peroneal tendon allograft at a 3-year follow-up interval. Indelicato et al. reported 93% of patients with a Lachman score of grade I or less and 78% having a negative pivot shift receiving a BPTB allograft at 27 months of follow-up. At a 3- to 5-year follow-up, Harner et al. found similar outcomes comparing allograft and BPTB autograft ACL reconstructions. Allografts tend to be used more often in ACL revisions and have provided a useful alternative to autograft options. However, longer follow-up studies have shown some downsides to using allograft for ACL reconstruction. For example, the MOON data analyses by Kaeding et al. indicated a 5.2 times greater risk of retear in allograft reconstructions than that in BPTB autografts (OR = 5.20; 95% CI: 2.60–10.44; P < .01). In addition, other MOON data analyses indicated the use of allograft in young patients as the only predictor for retear in a 2497-patient cohort.
The quality of allograft tissue is variable due to different company and processing standards. Allografts are harvested from cadaveric donors and treated using a variety of techniques including cryopreservation and gamma irradiation for sterilization. The sterilization process may affect the structural stability of the tissue. For example, >3 millirad (mrad) can result in weakening of the graft tissue, which limits sterilization techniques to using <3 mrad. Another concern in allograft tissue is the risk of infection. There have been three reported cases of disease transmission from ACL BPTB allografts, including HIV and Hepatitis C Virus (HCV). Allograft tissue is also slower to heal than autograft tissue and can be associated with an immune response because it is foreign to the patient’s body. Kaeding et al. demonstrated that allografts were associated with a four times higher failure rate than autografts for ACL reconstruction in the pediatric population (age range of 10–19), putting their efficacy into question for younger patients. Though these disadvantages exist, allograft remains a viable option for select ACL reconstructions in modern orthopedic practice, especially in patients older than 40 years, with multiple ligament reconstructions, and revision procedures. Kuechle et al. reported 96% of 28 patients over the age of 40 years who received an ACL allograft (either freeze-dried fascia lata or Achilles tendon) to have a 0–1 Lachman test, KT-1000 arthrometer readings within 5.5 mm of the contralateral knee, and 55% achieved the same or better preoperative activity level measured by IKDC classification.
There have been extensive studies evaluating the best graft choice for ACL reconstruction. In a meta-analysis of 21 studies including 1348 patients, Freedman et al. reported an overall failure rate of 1.9% in BPTB autografts compared with 4.9% in the hamstring autograft group. BPTB autografts remain the most widely used graft type to date. Patellar tendon autografts are recommended especially for younger, competitive athletes looking for a quicker return to sports. The bone-to-bone healing in BPTB may offer an advantage over other graft types in the young, active patient. Soft tissue grafts including the hamstring are also successful and have continued to grow in popularity as they offer high tensile strength, less donor-site morbidity, and consistent patient satisfaction. Allografts are used less frequently yet have proven to be successful in select patients. One study examined patients receiving soft tissue ACL allografts and reported that 79 of 84 patients returned to a desired activity level postoperatively. Allografts are successful in older, less active patients due to their lack of harvest morbidity and easier rehabilitation, with a longer healing time being more accepted by this demographic.
Large multicenter data sets such as the MOON cohort have provided valuable insight into the performance and outcomes of various ACL graft choices in the recent years. The MOON cohort analyses suggest a decreased risk of infection with BPTB autografts (0.3%) compared with hamstring autografts (1.3%) and all types of allografts (1.0%). It also concluded that there is a significantly increased risk of retear with allografts when compared with BPTB autografts. A MOON study of 2683 patients found that 3.2% of BPTB autografts, 4.6% of hamstring autografts, and 6.9% of allografts tore over the course of a 2-year follow-up. Though a higher percentage of hamstring grafts suffered retear than BPTB autografts, this did not reach statistical significance ( P = .12). Ultimately large database studies, such as those using the MOON cohort, provide objective data that surgeons can use when deciding what is the best ACL graft choice for the patient.
Synthetics and Xenograft
To date, synthetic graft and xenograft reconstructions have not had much success; however, they are still an area for further research in ACL graft technology. Legnani et al. provided a review of literature of artificial grafts in 2010, in which they recognized carbon grafts as the first synthetic graft form. The carbon grafts were eventually abandoned due to serious sequelae including early rupture, inflammatory synovitis, and deposition in the liver. Polytetrafluorethylene (PTFE) grafts followed and were implemented in clinical trials. After initial positive results, Paulos et al. demonstrated a 76% complication rate in PTFE grafts. Eventually in 1993 the PTFE graft was removed from the market after reports of complications, including inguinal lymph node involvement. The Dacron polyester graft, introduced in 1989, also reached clinical trials but was removed from the market due to various studies demonstrating rupture, increased knee osteoarthritis, and synovitis. Other synthetics such as the Kennedy ligament augmentation device have shown complication rates up to 63%, including increased infection risk.
A synthetic graft that has shown some promise is the Ligament Advanced Reinforcement System (LARS) ligament. The LARS ligament is composed of polyethylene terephthalate and contains sutures intended to allow growth inside the joint space. Lavoie et al. followed up 47 patients who underwent ACL reconstruction with the LARS ligament and found favorable postoperative results. Patients reported satisfaction with activities of daily living, recreational ability, and postoperative pain levels; however, patients on average did not reach preinjury activity levels. Jia et al. performed a 7-year follow-up in patients with a LARS ligament ACL graft and found an overall complication rate of 2.2%. They found decreased knee laxity measured by KT-1000 arthrometer, improved Lysholm scores, and an 87% return to sport. Given these outcomes, they deemed the LARS ligament ACL reconstruction graft to have a good prognosis. In another study comparing outcomes of artificial ligaments such as the LARS ligament, Jia et al. found the clinical outcomes and complication rates for synthetic grafts to be similar to those of BPTB and hamstring autograft ACL reconstructions.
Xenografts, or grafts from another species used for human implantation, are currently being investigated. A study by Zaffagnini et al. attempted to find a method to reduce the immunological reaction associated with xenograft placement in a different species. They have demonstrated that treatment with alpha-galactosidase can decrease the immune reaction associated with non–self-antigen alpha-Gal found in the porcine ligament. After a 2-year follow-up of three patients receiving the “Z-Lig device” which uses this concept, they observed a subjective improvement in Tegner scores, and one-legged hop tests postoperatively indicated functional return to a high level versus the unoperated knee at 12 and 24 months. Most importantly, blood tests of all three patients showed the absence of major anti-Gal and anti–non-Gal antibodies, demonstrating successful attenuation of the biological immune reaction to the foreign ligament. Further research into producing and testing a viable synthetic or xenograft ligament for ACL reconstruction is necessary before they may be considered a reliable graft option.
Biology of Healing after ACL Reconstruction
A successful ACL reconstruction not only relies on adequate graft choice, technique, and execution by the surgeon but also the biologic healing of the graft. Depending on the type of graft used, there are known processes of healing in which the patient’s body incorporates the new graft. Grafts that contain bone, such as the BPTB and quadriceps tendons, rely on bone-to-bone healing, specifically the incorporation of new bone into the tunnels created in the native femur and tibia. Other grafts, such as the hamstrings tendon, a soft tissue graft, rely on tendon-to-bone healing, a different biological healing process. Both methods have proven to be effective in ACL reconstruction, and it is important for the surgeon to recognize the various processes of healing so that they may facilitate the patient’s recovery.
Tendon-to-Bone Healing
Tendon-bone healing is paramount to the success of a reconstruction when an autograft or allograft is entirely of soft tissue. For example, the popular hamstring graft undergoes tendon-to-bone healing, which requires the healing of a newly introduced tendinous structure to the femoral and tibial bone tunnels. Cole et al. characterize the tendon-to-bone healing as either a compression or suspension fixation. Compression fixation secures the tendon at its bony insertion point, and suspension fixation uses fixation distant from the insertion site, such as the cortical periosteal or endosteal surface, cancellous, or cortical bone. Many researchers have shown that the native ACL insertion into bone can be broken down into four zones: (1) ligament, (2) unmineralized fibrocartilage, (3) mineralized fibrocartilage, and (4) bone. Genin et al. describe this transition, characterized by the increasing stiffness in collagen-fiber alignment and increased mineralization. In ACL reconstruction, the tendon graft is directly fixed into a bone tunnel creating a direct graft tendon-to-bone interface or bone-to-bone interface for BPTB and quadriceps grafts.
There are three phases of ACL graft soft tissue integration: (1) early, (2) proliferative, and (3) ligamentization. Cuti et al. describe an initial “early” phase, characterized by graft necrosis, hypocellularity, and blood vessel formation in an animal model. This phase typically lasts from weeks 1–4 postoperatively. The graft necrosis stimulates a cytokinetic and cellular response, drawing in neutrophils and macrophages via the release of tumor necrosis factor-a, interleukin 1-b, and interleukin 6. These cells work to eliminate waste and continue to release cytokines to facilitate the inflammatory response. The formation of granulation tissue fills the gap between the bone and the graft and is rich in vascular endothelial growth factor (VEGF) and fibroblast growth factor, promoting revascularization and recruitment of fibroblasts, respectively.
The “proliferative” phase of healing is characterized by increased cellularity during weeks 4–12 and includes the presence of mesenchymal stem cells that help to facilitate graft remodeling. Scheffler et al. described an invasion of myofibroblasts that takes place toward the end of the proliferative phase. The fibroblasts replace granulation tissue and exert tension on the cellular and extracellular matrix, restoring the “ligament-like” tension during the healing process. As the healing process continues, macrophages release transforming growth factor-beta (TGF-β), facilitating the creation of a fibrovascular scar that connects the graft to the native bone. These perpendicular type III collagen fibers are called “Sharpey-like fibers.” These bundles function to resist shear stress placed on the graft during the early phase of rehabilitation. At week four of a rabbit study, Liu et al. noted a dense connective tissue matrix joining the bone to the soft tissue hallucis longus tendon graft, and at 6 weeks, there was a fibroblastic cellularity with longitudinal collagen organization. At 8 weeks postoperatively in rabbit models, Kanazawa et al. found that type III collagen is deposited around the fibroblast-like cells that move toward the center of the healing semitendinosus tendon. Throughout the proliferative phase, bone tunnel remodeling also takes place, characterized by osteoblast activity producing new bone to fill the void of the tunnels.
Many studies have examined the mechanical stability of ACL grafts postoperatively. At weeks 6–8 postoperatively, several studies show that the graft is at its weakest mechanical point. Some suggest that this decreased mechanical stability can be attributed to the disorganized collagen network that is laid down during the healing process. This collagen network starts to become organized late in the healing process, when the graft begins to resemble a native ACL. At this point in rehabilitation, the graft must be loaded carefully to avoid compromise, but appropriate loading is required to ensure the continuation of graft integrity.
The final phase of ACL graft healing involves “ligamentization” of the tendon. At approximately 12 weeks, the graft finally starts to resemble a native ACL in strength. It is important to note, however, that the new ACL graft never truly replicates a native ACL because it has a different histologic and morphologic appearance. Hypercellularity and vascularization during the proliferative phase decreases to levels similar to those of the native ACL by 6–12 months in animal models. In a biochemical study by Marumo et al., longitudinally oriented collagen fibers and spindle-shaped cells were observed at 4–6 months postoperatively. They believe that the ligamentization process can continue for up to 1 year. As Cole et al. noted, the entire healing process plateaus at approximately 6 months postoperatively. A small margin void of bridging collagen or mineralization between tendon and bone can even remain. Suspensory fixation methods can even exhibit granulation tissue and irregular bone without collagen ingrowth between the graft and native bone for up to 6–14 months.
Bone-to-Bone Healing
Compared with soft tissue grafts, ACL grafts containing bone heal by a different mechanism, similar to fracture healing. Grafts that undergo this type of healing include BPTB and quadriceps because they are harvested with a bone plug. Direct contact between the bone tunnels in the tibia/femur with the ACL graft bone plug provides a site for bone-to-bone healing. Once the bone plug and native bone are in contact, the formation of new bone begins. Inflammatory cells, mesenchymal stem cells (MSCs), and chemotactic agents are abundant in the bone-to-bone interface. Granulation tissue forms and macrophages remove cellular debris as the osteoclasts continue to resorb bone. Osteoblasts eventually lay down osteoid, which is then mineralized to form new bone. This process can take anywhere from 6 to 12 months after ACL reconstruction.
Tomita et al. performed a randomized trial observing the intraosseous graft healing between a doubled flexor digitorum superficialis and a BPTB graft in canine ACL reconstructions. At 3 weeks postoperatively from BPTB autografts, they observed the anterior portion of the bone plug and tunnel interface filled with granulation tissue, whereas the posterior bone plug was in direct contact with the bone tunnel wall. On high-power magnification, they noticed few collagen fibers surrounding the intraosseous tendon portion, osteocyte necrosis throughout the bone plug (excluding the superficial layer), and limited change in the tendon-to-bone junction of the graft itself. At 6 weeks, the granulation tissue in the anterior gap remained, the posterior gap demonstrated new bone formation, and Sharpey’s fibers began to bridge the space between the bony wall and the intraosseous tendon portion of the graft. Also, the bone plug of the BPTB graft showed new bone formation and anchoring to the bone tunnel wall. A decrease in cartilaginous cells in the bone-to-tendon interface was also noted. At 12 weeks, more bony integration was seen in the posterior gap, perpendicular collagen fibers were increased in number, and granulation tissue in the anterior gap remained at levels similar to soft tissue ACL grafts. At 3 and 6 months postoperatively, the BPTB graft demonstrated a higher ultimate failure load than the flexor tendon graft, demonstrating its potentially quicker healing potential and early strength.
Biologic Augmentation in ACL Reconstruction
The use of biologic augmentation in ACL reconstruction is a developing and controversial topic in orthopedic surgery. The most commonly studied biologic agent is platelet-rich plasma (PRP), an autologous blood product with increased concentration of platelets compared with whole blood. Platelets have been isolated as a valuable biologic agent due to the inherent growth factors known to stimulate tissue healing. Another novel biologic agent undergoing orthopedic research is stem cells, primarily MSCs acquired from bone marrow adipose. There are numerous in vitro and animal studies on this topic, but clinical evidence is still lacking, as the concept is relatively new.
Most clinical studies regarding PRP in ACL reconstruction have focused on clinical outcomes, bone tunnel enlargement, the graft-bone interface, and ACL graft maturation. Functional scores have primarily been assessed by physical examination, KT-1000/2000 testing, the Lysholm scale, Western Ontario and McMaster Universities Osteoarthritis Index (WOMAC) stiffness scale, and so forth. Advanced imaging by computed tomography (CT) and magnetic resonance imaging (MRI) has been used to evaluate bone tunnels, the graft-bone interface, and ACL graft maturation.
Clinical outcomes have been measured using various methods including functional scores, objective examination findings, and in some studies, return to sports. Del Torto et al. noted no significant difference in objective IKDC scores between patients with a platelet-rich fibrin matrix added to the hamstring autograft versus the control. However, they did find a significant difference in subjective IKDC scores. Because the patients were not blinded, this could be due to patient-reporting bias. Valenti et al. also noted no difference in objective IKDC scores when comparing platelet-rich growth factor (PRGF) using a single-spin method without leukocytes, a PRP spin with leukocytes, and a control. Their study also found no difference in objective functional tests including knee range of motion, muscle torque, and KT-1000. There was, however, a notable difference in decreased postoperative effusion for the PRFG group, which has been demonstrated in other studies as well. Interestingly, Darabos et al. used an intraarticular injection of autologous conditioned serum consisting of cytokines and growth factors on the day of ACL surgery (day 0), day 1, day 6, and day 10, and they also found a decrease in postoperative effusion with an associated decreased WOMAC stiffness subscore after 1 year compared to placebo patients. The authors attributed this to the known anti-inflammatory effects of cytokines and growth factors that are found in PRP. This study also found that interleukin-1B synovial concentration, a marker of inflammation, was lower on postoperative day 10 for the ASC-injected patients than that for placebo-injected patients, further supporting an anti-inflammatory effect of using a platelet adjunct. One study using platelet-leukocyte gel applied to the hamstring autograft noted better anterioposterior stability using KT-2000 arthrometer at the 6-month evaluation. Conversely, other studies have negated this finding, claiming that there is no difference in stability as measured by the Lachman test or KT-1000. At this time, only data for a short-term follow-up of 2 years or less exist. Therefore, more studies are needed to understand whether the use of PRP provides a long-term difference in clinical outcomes for ACL reconstruction.
Can We Improve Graft-to-Bone Healing?
An important aspect in the success of ACL reconstruction is the incorporation of the graft into the native knee during healing. The graft is placed through femoral and tibial bone tunnels during surgery. Poor graft-bone healing can cause early postoperative failure of the reconstruction.
Bone tunnel widening
Bone tunnel widening is a phenomenon seen after ACL reconstruction. Although this may not be significant in clinical outcomes, widened bone tunnels add complexity to revision surgery, sometimes requiring staged operations. Some believe it occurs more in hamstring than patellar autografts and with extracortical fixation due to mechanical etiologies. Others believe that it is due to biologic factors activated by drilling through bone to create tunnels for graft insertion. Therefore biologic agents have been considered as a means to decrease bone tunnel enlargement postoperatively. Mirzatoloogei et al. found no difference in bone tunnel widening at 3 months postoperatively after hamstring autograft with PRP application to both femoral and tibial tunnels. Vadala et al. agreed with this finding using similar operative and biologic techniques, finding no difference in tunnel enlargement. However, a few studies did find a significant difference with decreased tunnel enlargement after application of PRP measured by CT at approximately 1 year postoperatively. It is thought that improved graft-bone integration may decrease bone tunnel enlargement. Therefore the size of postoperative bone tunnels may be a prognostic sign of better graft incorporation. There have been no studies that correlate bone tunnel widening directly to worse outcomes, but it is a significant factor when considering ACL revision for graft failure.
Orrego et al. did a randomized control study comparing the use of platelet concentrate placed onto the hamstring autograft versus using a bone plug harvested from the tibial tunnel and placed by interference fit in the femoral tunnel, and the combination of both on the effect of bone tunnel widening as well as graft-bone interface. They demonstrated a significant difference in decreased bone tunnel widening using the bone plug, but no difference with platelet concentrate or the combination of both was found. It is unclear whether this is due to a decrease in the number of cytokines causing osteolysis of the bone or a reduction in mechanical motion which may attribute to tunnel widening. MRI was also used to detect signal intensity around the graft, which characterizes tendon integration. They found no difference between any groups at 6 months. This was consistent with other studies that found no difference in postoperative MRIs of ACL reconstructions using platelet augmentation versus no augmentation.
Vascularity
Another principal factor in graft-bone integration is revascularization during the healing phase. Vogrin et al. did a study focusing on the revascularity of the graft-bone interface as well as intraarticular graft substance using platelet gel with the hamstring autograft. He noted that there was a significant increase in vascularization at the osteoligamentous junction at 4–6 weeks of the ACL grafts with platelet augmentation compared with the control but with a relatively decreased vascularity at 10–12 weeks postoperatively. He attributed this change to the replacement of vascular tissue with hypovascular collagenous scar tissue in the zone between the graft and the bone tunnels, which suggests normal graft healing. The control group had a slightly higher vascularity at the second follow-up point than that at the first, for which they concluded a slower healing process in the control group than in the augmented group. There was no difference in healing of the intraarticular segment of the graft. Another study by Rupreht et al. supported a similar finding with increased vascularity of the tibial tunnel. They found that locally applied PRP gel to the hamstring tendon autograft reduced tendon-bone interface edema in the first postoperative month on MRI. They also found an increase in vascular density and microvessel permeability in the tibial tunnel in the first couple of months postoperatively. These findings of decreased edema and increased vascularity of the tibial tunnel support the use of PRP on ACL reconstruction to aid in graft incorporation.
Stem cells
Stem cells derived from the bone marrow are another biologic agent with healing potential that is a newer concept and much less studied than PRP. MSCs are multipotent cells, which have the ability to differentiate into several different musculoskeletal structures such as cartilage, bone, muscle, tendon, and ligament. For this reason, they are under investigation for their healing potential in ACL reconstruction. As of right now, there are many in vitro and animal studies published with various promising results; however, very little clinical data exist on this topic.
There have been several animal studies focusing on stem cell augmentation for ACL reconstruction. Lim et al. used bone marrow stem cells (BMSCs) to coat hamstring autografts during rabbit ACL reconstruction and found a greater number of chondrocytes at the tendon-bone junction with a subsequent mature zone of cartilage than the control with only mature scar tissue. Other animal studies have shown similar benefits, with the administration of BMSCs demonstrating increased collagen expression and decreased catabolic proteins after ACL reconstruction, which also signifies improved tendon-bone healing. In addition to these histologic discoveries, there are also animal studies showing a positive effect on mechanical strength by testing load to failure of BMSC-enhanced grafts compared with the control grafts. These animal studies may represent superior healing potential of ACL grafts with BMSC augmentation, but clinical trials are needed to further validate these results.
Silva et al. attempted to use adult noncultivated bone marrow stem cells to improve the healing time of the tendon-bone interface in the femoral tunnel during hamstring allograft ACL reconstruction. They performed an anterior iliac bone crest aspiration of the patient during surgery in a commercially available kit to isolate the stem cells. Half of the concentrate was injected into the graft, and the other half was injected into the femoral tunnel. MRIs were obtained at 3 months and measured for signal intensity at the graft-bone interface. They found no difference in graft incorporation compared with the control. Although BMSCs have been shown to accelerate the healing of the graft-bone junction in animal models, clinical studies to date have been unable to reproduce these results.
Can We Improve the Biology of the Graft?
Improving the biology of an ACL graft is a topic of interest, particularly in graft remodeling and maturation. Using biologic augmentation to expedite graft maturation would allow for quicker healing and potentially improve clinical outcomes while decreasing the risk of early failure. Orrego et al. not only studied bone tunnel widening as previously mentioned but also investigated graft maturation by assessing signal intensity within the graft, with a low-intensity signal indicating graft maturation. At 6 months of follow-up, there was a significant difference in graft maturation in the platelet concentrate group compared with the control group. Another study injected PRP after surgical portals were closed and compared the MRIs with those of patients who did not receive the injection. They found increased maturation on MRI signal intensity at 4 and 6 months, but no statistical significance at 12 months. Radice et al. applied PRP gel to BPTB autografts and measured maturation by MRI heterogeneity. This study reported a shortened time by 48% (179 days) to reach graft maturation compared with the control (369 days). One could infer that this means potential graft healing in almost half of the time with PRP as an adjunct. Although MRI is a practical method to obtain objective data on graft maturation, there may be some interobserver error. Furthermore, there are several studies that claim there is no difference in graft maturation when evaluated by MRI. Rather than relying on MRI studies to evaluate graft maturation, Sanchez et al. published a study of second-look arthroscopy at 6–24 months after surgery with direct evaluation and histologic analysis of previous ACL grafts with and without PRGFs. They compared graft thickness and apparent tension, which were reviewed by blinded observers, and found that the ACL grafts treated with biologic augmentation rated higher, but this did not reach statistical significance. The histology was evaluated using the Ligament Tissue Maturity Index of Murray et al. and found the grafts with PRGF to have more mature tissue. Interestingly, they noticed a new connective tissue formed around the graft, which occurred more frequently in grafts augmented by PRGF. This highly vascular envelope became denser over time until it was nearly indistinguishable from the original graft. All these patients required a second-look surgery for other intraarticular pathology, which is not part of standard ACL reconstruction postoperative care. Therefore this study had a limited number of patients, and they may not be generalizable to ACL patients without postoperative pathology. A second-look arthroscopic surgery does provide the most direct and objective analysis; therefore this information is important. However, graft maturation has not been directly related to clinical outcomes in any of these studies and therefore may be clinically insignificant.
Undoubtedly, there is still significant controversy on the topic of biologic agent augmentation with ACL reconstruction. The results of clinical outcomes, graft-to-bone healing, and ACL graft biology are inconclusive at this time. However, using these biologic agents has not shown to cause harm; therefore more research is warranted to better define the beneficial qualities of biologic augmentation in ACL reconstruction.
Can We Improve Healing of Partial Tears?
Partial ACL tears represent only about 10%–28% of ACL tear patients, yet there are ongoing discussions in the orthopedic community regarding the treatment of incomplete tears. When compared with other ligaments of the knee, the ACL has poor ability for primary healing, usually requiring intervention to facilitate a full recovery. As described by Dallo et al., the synovial fluid of the knee joint has inhibitory properties preventing fibrin-platelet clot formation and fibroblast recruitment, which results in poor primary healing potential. In an attempt to overcome the poor healing potential of partial ACL tears, the efficacy and viability of biologic augmentation is a topic of growing interest. Though not yet popular in 2018, biologics may offer a potential option for treatment of incomplete ACL tears in the future. Options for augmentation include PRP injection, biologic scaffolds, and stem cell therapy.
Platelet-rich plasma
The use of PRP is common in other orthopedic procedures, and although some research exists regarding the use of PRP in combination with reconstruction procedures, there is limited literature regarding its sole efficacy in partial ACL repairs. PRP is known to contain various growth factors that help to facilitate the healing and blood clot formation process, including TGF-β1, platelet-derived growth factor (PDGF), VEGF, and basic fibroblast growth factor. The PDGF and TGF-β1 specifically facilitate recruitment of fibroblasts and collagen growth, which enhances the healing of damaged connective tissue. Seijas et al. described a study involving 19 patients with previous partial ACL tears. Patients were assessed via diagnostic arthroscopy, and a senior surgeon with experience in ligamentoplasty assessed the integrity of the remnant ACL bundle. All 19 patients demonstrated a complete rupture of the anteromedial bundle with an intact posterolateral bundle and therefore were included in the study. Four milliliters of PRGF was injected in the proximal and middle portion of the intact bundle, as well as in the articular space. They found that 16 patients who received the injection returned to their previous level of playing sports on national and international soccer teams at an average of 12.33 weeks for Tegner 10 and 16.2 weeks for Tegner 9 patients. At the 1-year MRI follow-up, complete ligamentization was seen in all cases, and at the 2-year follow-up, all patients had no sign of instability. In a canine model by Bozynski et al., subjects with partial ACL tears treated with PRP had better postoperative outcomes in terms of pain, effusions, function, and range of motion than groups treated with Non-steroidal anti-inflammatory drugs (NSAIDs) only. These differences, however, were not statistically significant. In a similar study by Cook et al., canines with partially transected ACLs as well as meniscal lesions were treated with leukoreduced PRP injections and observed alongside a control group treated with saline injections. Within 1 week, decreased pain and increased range of motion was noted in the PRP group, and within 5 weeks, there was an improvement in subject lameness, kinetics, and function. However, at the 6-month period, the differences in outcomes when compared with the control group were not statistically significant. Owing to limited research and lack of statistical significance, there is not enough evidence to recommend the treatment of incomplete ACL tears with PRP injections alone.
Murray et al. performed a porcine-based experiment in which the animal ACLs were completely transected and treated with PRP injections. They observed no benefit of the PRP injections, hypothesizing that the formed clot dissolves in the joint space precluding activity at the site of healing. This is likely due to the intraarticular presence of urokinase plasminogen activator which increases plasmin, leading to fibrin degredation. Ultimately, this finding has steered the field toward exploring the option of PRP combined with biologic scaffolds to hold the PRP material near the intended site of healing.
PRP plus collagen scaffold
The use of PRP injections in combination with a biologic scaffold to hold the injection to the site of healing has shown some promising outcomes in its infancy. Dunn et al. initially described a collagen-fiber scaffold used for ACL reconstruction procedures that allows better fibroblast attachment and proliferation as well as increased collagen synthesis. This scaffold provides a location for fibroblasts to proliferate and lay down collagen fibers near the site of injury, thereby improving healing directly near the site of the injured ACL. Combining targeted healing with the enhanced regenerative potential of PRP injections has shown potential to improve the healing of incomplete tears. Vavken et al. described the combination of a collagen scaffold with PRP. They demonstrated that fibroblasts respond to platelet concentrate, leading to increased proliferation and collagen production. In a canine model, Murray et al. found that partial central ACL defects treated with a collagen scaffold and platelets resulted in improved biomechanical properties after healing compared to the no treatment group. Interestingly, they observed similarities between the healing of a partial ACL tears treated with collagen platelets with scaffolding and a patellar tendon graft on a histologic level. Studies such as these have demonstrated that partial ACL tear healing is more efficient when PRP and a biologic scaffold are used in tandem rather than separately. In 2016, Murray et al. described the bridge-enhanced ACL repair procedure (BEAR procedure). The technique involves suture repair of the ligament combined with a bioactive scaffold that fills in the gap between the torn ligament fragments. The BEAR scaffold consists of extracellular matrix proteins including collagen from bovine tissue which has been treated to remove DNA content to less than 50 ng per milligram of scaffold. It is a highly hydrophilic material, allowing absorption of up to 5 times its weight in fluid. They predicted that the implantation of the graft into the intraarticular space would not result in significant inflammation or infection and that it would lead to similar early postoperative outcomes compared with ACL reconstruction using an autologous hamstring graft. The BEAR scaffold is not cross-linked similar to other biologic scaffolds. Murray et al. believed this would allow cells to more easily permeate and implant into the scaffold. In turn, the increased permeability leads to improved resorption of the material, therefore decreasing inflammatory immune reactions. The BEAR scaffold is also highly processed to remove any remaining bovine DNA that can serve as a potential immune trigger, which was demonstrated in preclinical testing. In their nonrandomized study, 10 patients received the BEAR treatment, and 10 received standard ACL reconstruction with hamstring autograft. Results showed similar outcomes among the two groups using measurements such as effusion, pain, Lachman tests, and MRI analysis of ligament continuity. At 3 months, the BEAR group showed better hamstring strength than the hamstring autograft reconstruction group (mean ± standard deviation: 77.9 ± 14.6% vs. 55.9 ± 7.8% of the contralateral side; P < .001). Success of the BEAR technique in initial testing may demonstrate its readiness for further testing in a larger cohort of patients. A potential downside to the experiment by Murray et al. is that only patients with greater than 50% of the ACL tibial remnant were included in the study. This makes it difficult to extrapolate successful results to more severe tissue resorption or patients with less than 50% of the ACL tibial remnant intact.
Murray et al. went one step further to consider the addition of thrombin to the PRP-collagen scaffold for further testing. In vivo, thrombin improves the healing process by increasing fibroblast growth and collagen synthesis. They found that a low concentration of thrombin added to the PRP-collagen scaffold can aid in recruitment of inflammatory cells but was not beneficial at high concentrations. Further research is required to identify the benefit of adding low concentrations of thrombin to a PRP-collagen scaffold to aid in the repair of partial ACL tears.
Though studies demonstrating the improved healing of partial ACL tears using PRP and collagen scaffolds are promising in canine models, further studies are needed to investigate ACL strength, long-term outcomes, and viability/applicability in human trials.
Stem cell therapy
The use of MSCs in orthopedic procedures has also stimulated interest in the research field. MSCs are derived from various sources, such as adipose, bone marrow, cartilage, periosteum, and muscle. Their capability to differentiate into various cell types makes them a good candidate for therapeutic use, especially to improve healing. MSCs are capable of regenerating themselves, as well as developing into cells such as chondroblasts and osteoblasts, which are beneficial in the bone-healing process. Kanaya et al. performed a rodent study in which fluorescently tagged MSCs from the same rodent species were injected into the articular space surrounding a partially torn ACL. Results were compared to a control group where injections consisted of only buffered saline. They found that at 2 and 4 weeks after injection, the group receiving MSCs showed fluorescently tagged cells located at the partially torn ACL surface. Histologically, the group that received MSCs received a higher score, and at 4 weeks, the failure load was higher than that of the non-MSC group. In a similar rat study, Oe et al. created three test groups of partially transected rat ACLs. One group received saline injections, one received cultured MSCs, and the third received fresh bone marrow cells (BMCs). They found that at 4 weeks, both the treatment groups showed cell migration to the site of healing and both appeared histologically normal. The BMC group showed more spindle cell formation, higher tensile strength, and more TGF-β in the joint space than the saline group. These findings suggest that the use of fresh BMCs may improve the healing of partial ACL tears in animal models.
To our knowledge, the only existing human study that investigated BMSC injections for partial ACL tears was performed by Centeno et al. in 2015. They evaluated grade 1–3 ACL tears (with grades 1 and 2 indicating partial tears) that had less than 1 cm ligament retraction. Ten patients were treated with fluoroscopically guided bone marrow concentrate PRP injections and assessed by MRI. Comparing magnetic resonance images from before and after the injection for each subject, they were able to assess ligament integrity using specific computer software and five MRI objective outcomes. Seven out of 10 patients had improved outcomes based on four out of five of their MRI measurements. Eight patients subjectively reported at least 70% improvement after the injection, and the mean improvement reported was 86.7%. Based on the findings of this study, it is possible that BMCs may be beneficial to the treatment of partial ACL tears with less than 1 cm ligament retraction, though additional human subject testing is warranted.
Future
The future of biologic augmentation in ligament reconstruction shows promise. Currently, there are several animal studies, but few human trials, involving biologic augmentation for ACL reconstruction. More investigation of the biological treatment options, long-term effects of these treatments, and side-by-side comparisons with traditional treatment methods would provide crucial insight into the future of ACL reconstruction. To better understand the benefit of this new technology, more clinical trials are needed.
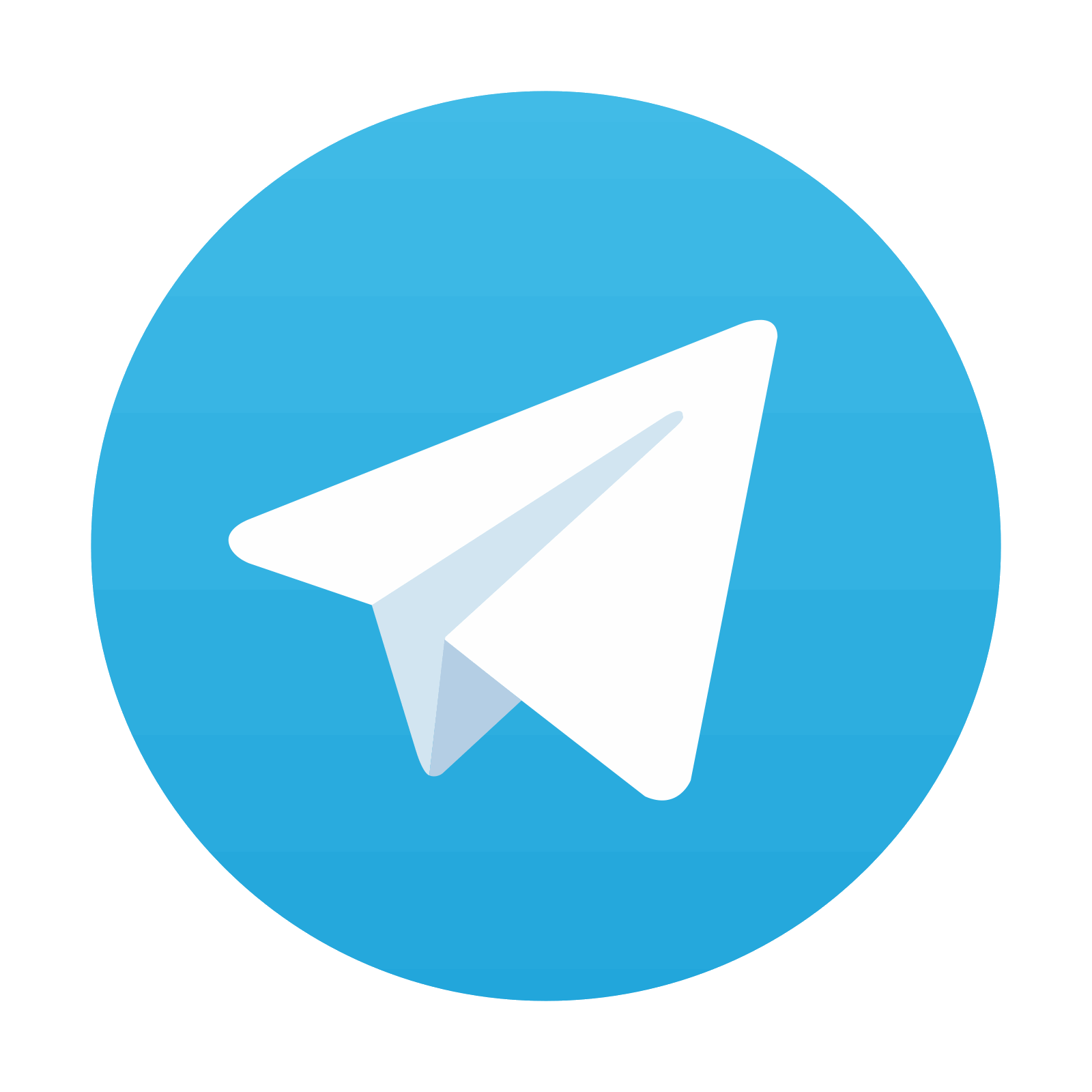
Stay updated, free articles. Join our Telegram channel
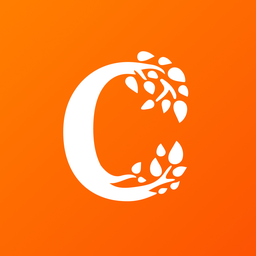
Full access? Get Clinical Tree
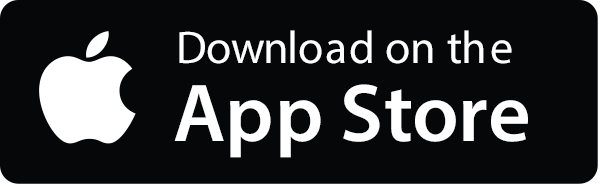
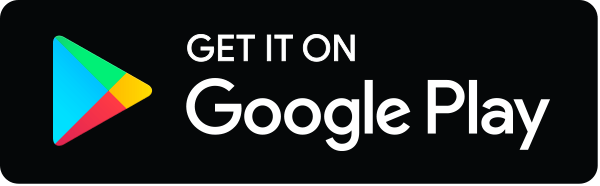