Abstract
Peripheral nerve injuries are known to have devastating impact on a patient’s quality of life. These injuries can occur after high-impact activities, traumatic injuries, or motor vehicle accidents. When a nerve is transected or crushed, it encounters roadblocks that must be overcome for efficient and effective neural regeneration. Although microsurgical reconstruction offers hope for improved results after surgery, surgical manipulations alone have not optimized the outcomes. Tensionless, primary repair is the gold standard for nerve injuries without gaps, whereas injuries with segmental defects challenge standard surgical techniques for nerve repair. As such, modalities such as structural guides and luminal additives are and continue to be developed in an attempt to improve outcomes. Furthermore, research has focused on accelerating the process of regeneration after injury through pharmacological agents, implantation of stem cells, and gene therapy, all for the ultimate purpose of better functional recovery at the end. In this chapter we review the current literature relevant to the utilization of available biologic augments in peripheral nerve repair.
Keywords
Biologic augments, Nerve allografts, Nerve defect, Nerve regeneration, Peripheral nerve injury, Synthetic conduits
Introduction
Peripheral nerve injuries remain a main source of morbidity and disability. Peripheral nerve injuries have life-altering impacts on the patients who suffer years of uncertainty while waiting for some level of recovery. Patients may be left with devastating sensory and motor deficits such as limb numbness, dysethesias, paralysis, and neuropathic pain which renders them disabled. The management of nerve injuries is multifactorial including the location of the injury, the type of injury, the size of segmental nerve defect, the timing of injury presentation, and accompanying soft tissue injury. Nerve injuries lead to both physiological and histopathological changes to the nerve and its surrounding soft tissue, including demyelination, degeneration, remyelination, and regeneration. Despite the permissive growth environment of the peripheral nervous system (PNS), major human nerve injuries have very limited potential for spontaneous recovery. Although there has been significant amount of research addressing the molecular biology of nerve injury and numerous surgical advances detailing peripheral nerve repair, the injured limb still cannot be restored to normal function. Unfortunately, optimization of outcomes has plateaued with surgical manipulation alone as issues with the rate of regeneration, specificity of regeneration, segmental nerve defects, and degeneration of target end organ remain unaddressed by current practices. Tension-free primary repair is the gold standard for peripheral nerve injuries that do not have gaps. Nerve injuries with gaps present a greater challenge for the reconstructive surgeons. Faced with this predicament, surgeons have been searching for alternative techniques to allow bridging of gaps, as well as tensionless nerve repair. The role of biologic augments in the repair of peripheral nerve injuries has long been studied in both animal models and humans to address this issue. It is, therefore, critical that we are made aware of the appropriate applications and the limitations of these tools to understand how we can promote an effective regenerative environment for damaged nerves. Adapted from our recent review, we include discussions regarding microanatomy, nerve response to injury, as well as limitations of peripheral nerve regeneration, as these topics provide invaluable information to further understand the role of biologic augmentation in peripheral nerve repair and reconstruction.
Microanatomy
Peripheral nerves are heterogeneous composite structures that are comprised of neurons, Schwann cells (SCs), macrophages, and fibroblasts. The neuron is a polarized cell that forms the foundation of the nerve and consists of dendrites, the cell body, and a single axon. Axons project toward their sites of innervation to form synapses with their target end organs. If the axonal diameter is greater than or equal to 1 μm, each SC will wrap its plasma membrane around one single region of an axon to form myelin. SCs produce myelin to encapsulate the axon and aid in action potential transmission as myelin allows for fast and efficient conduction and propagation of an action potential down an axon. The blood supply to the nerve is a complex vascular plexus formed from anastomoses of epineural, perineural, and endoneurial plexi, as well as segmental blood supply derived from a number of nutrient arteries. It is apparent that the blood supply to the nerve is fragile and may be disrupted due to trauma or tension during nerve repair. In addition, peripheral nerves have connective tissue layers to provide strength and protection to the nerve: (1) the epineurium, (2) perineurium, and (3) endoneurium. It is crucial to recognize that all surgical interventions are strictly directed at these connective tissue layers, whereas the axon and SCs must respond to injury and regenerate via their inherent biology.
Nerve Response to Injury
In contrast to chronic nerve injuries that are SC driven, acute nerve injuries are axonally mediated with Wallerian degeneration being initiated with granular disintegration of the axonal cytoskeleton. Within 48 hours after injury to the nerve, SCs break down myelin and phagocytose debris from the axon in the distal stump. Macrophages are then recruited to the area and start releasing growth factors that in turn encourage SC and fibroblast proliferation. SCs begin the reparative process by forming longitudinal bands of Bungner, which are essentially growth-promoting conduits for regenerating axons. Injection of predifferentiated SCs near injured nerves has been shown to aid remyelination in regenerating axons, reduce percent myelin debris, and improve functional recovery in rodents. At the tip of the regenerating axon is the growth cone, which is composed of cellular matrix from which fingerlike projections called filopodia extrude to explore the microenvironment. Proteases are released from the growth cone to clear a path toward a target organ, which is heavily influenced by different factors. After injury, SCs upregulate neurotrophic factors nerve growth factor (NGF) and brain-derived growth factor (BDNF), as well as their corresponding receptors in the distal stumps. This increase in expression of NGF and its low-density receptors is believed to promote extensive proliferation and migration of SCs and mainly affects properties of sensory neurons. BDNF levels are also increased and are postulated to act as an anterograde trophic messenger under the influence of NGF. Ciliary neuronotrophic factor (CNTF) is a neuronotrophic factor that is believed to affect survival and regeneration of motoneurons and is found to be reduced significantly in the SCs of the distal stump, with this reduction extending to the neuromuscular junction. Furthermore, there is increased retrograde axonal transport of CNTF after nerve injury. Neurite-promoting factors, such as laminin and fibronectin, and matrix-forming precursors, such as fibrinogen, are all synthesized in response to nerve injury.
In addition to the release of trophic factors, tubulin deacetylation is an important factor leading to decreased stability of microtubulin, which is required for axonal integrity and regeneration. Calcium-dependent activation of the histone deacetylases HDAC5 and HDAC6, in particular, leads to tubulin degeneration likely playing a role in inhibiting axonal regeneration after peripheral nerve injuries. The interaction between axons and SCs has also emerged as an important regulator of PNS development and regeneration. Fleming et al. identified that the receptor tyrosine kinase Ret genetically interacts with Er81 to control Nrg1-Ig in promoting the formation of Pacinian corpuscles. Taken together, these factors have the potential to promote regeneration; provide signaling for cell survival, neuronal differentiation, and proliferation; and influence synaptic function.
After a nerve injury, PNS neurons upregulate a number of regeneration-associated genes that may have direct role in neurite outgrowth. For example, the overexpression of transcription factor Activating transcription factor 3 (ATF-3) has been shown to promote neurite outgrowth after peripheral nerve injury. In their animal study, Bomze et al. concluded that growth-associated protein 43 and cytoskeleton-associated protein 23 were expressed after nerve injury and, together, were able to induce dramatic increase in the number of regenerated axons. Pathways associated with increased regeneration after a nerve injury have also been identified. The ERK pathway was shown to mediate axonal elongation, with the kinases Extracellular signal regulated kinase (ERK) and Akt promoting regeneration after axonal injury. After nerve injury, the cytokine interleukin-6 has been shown to work through the JAK-STAT3 pathway to overcome the inhibitory molecules and allow for axonal regeneration. In addition to pathways that are involved in axonal regeneration, there are also pathways that have been associated with inhibition of axonal regeneration. The small GTPase Rho signaling pathway, for instance, has a role in cytoskeletal reorganization and cell motility. Studies have shown that activation of Rho results to collapse of growth cones, and inhibiting Rho pathway allows for contractility and promotes neurite outgrowth.
Limitations of Peripheral Nerve Regeneration
Despite the promise of improved functional recovery, results are still limited as detailed by these studies with all currently available techniques. Outcomes of nerve repair after a traumatic injury are commonly influenced by factors outside of the control of the surgeon such as the age of the patient, location of injury, and timing of injury presentation and nerve repair. As initially reported by Sunderland and subsequently by many others, nerve reconstruction outcomes are better with younger patients, early repairs, repairs of single function nerves, distal injuries, and short nerve grafts. There are numerous challenges facing nerve repair, and optimization of outcomes has plateaued with surgical manipulations alone, offering limited capacity to effect true functional neural regeneration. The ultimate repair and regeneration is a complex biological process that we are just beginning to understand through clinical experiences as well as animal experimental studies. Rate of regeneration, specificity of regeneration, segmental nerve deficits, and degeneration of the target end organ are challenges that need to be overcome to achieve meaningful functional recovery ( Fig. 14.1 ).

Rate of Regeneration
In adults, it is well accepted that neural regeneration occurs at a slow rate of about 1 mm per day. The rate of regeneration can be monitored with a present advancing Tinel sign as it progresses from proximal to distal. When a nerve is injured, the nerve must grow over substantial distances and traverse over scar and fibrous changes in its environment to reach its target. For example, a brachial plexus injury may involve distances of up to 1 m and may require up to 3 years for regenerating axons to reach the hand muscles.
Many factors can limit and influence the rate of nerve regeneration. The type of nerve injury can influence the probability of successful regeneration. A crush nerve lesion for instance has a continuous basal lamina structure that provides guidance for regenerating nerve. After axotomy, the nerve sheath discontinuity impedes reinnervation and can lead to neuroma formation. Animal studies have also shown that production of neurotrophic factors in the distal segments of a nerve gradually decreases to the point where sufficient levels are not present to support nerve growth, preventing fast regeneration. Furthermore, this nongrowth permissive state promotes axonal retraction and “wandering axons” which is thought to prevent regeneration to the neuromuscular junction.
Specificity of Regeneration
Axonal misdirection is believed to play a significant role in poor functional recovery after severe nerve injuries. Nerve injuries induce rapid axonal sprouting so as to facilitate anterograde, target directed axonal regeneration. Moreover, the degenerating distal nerve segment has growth-promoting potential and may enhance the specificity of regeneration. Quantitative retrograde labeling technique has been used to define the number of regenerating motoneurons and the specificity of their peripheral connections. It has been shown that motor nerves tend to reinnervate motor pathways. Even in injured mixed sensory-motor nerves, it was observed that motor axons preferentially reinnervate motor pathways. This involves recognition molecules of the L2/Human Natural Killer-1 (HNK-1) family that are detectable in ventral spinal roots and motor axons but not in dorsal root or sensory cutaneous nerves. As a nerve tries to regenerate, motor axons were found to explore different pathways by sending out collateral branches. Specificity of pathway regeneration is subsequently gained by “pruning off” those collaterals that have grown into inappropriate nerve branch. After injury, muscle fibers previously belonging to a specific motor unit will likely be innervated by a different motor axon. This mismatch between central commands to motor neurons and the actual distribution of muscle fibers innervated by those motor neurons contributes to unsuccessful functional recovery, as well as sensorimotor disturbances. Furthermore, after injury, some axons grow in various directions and compete for innervation, which will lead to loss of prelesional innervation selectivity. A study visualizing regenerating axons reported axons exposed to as many as 150 different potential distal pathways. Although preferential motor reinnervation has been detailed in animals, it remains unclear as to what level this occurs in the human condition.
Segmental Nerve Defects
Intuitively, regeneration over a segmental gap is quite challenging and has been demonstrated with animal studies as epineural neurorrhaphy without a gap showed better nerve regeneration when compared with neurorrhaphy with a gap. Functional restoration after a nerve injury requires the growth of axons over the distance between the lesion and the end target. After injury, fibrosis and edema cause nerve fibers to lose their elasticity and extensibility, as well as a certain amount of retraction. If a nerve tissue is destroyed, the fascicular pattern of the proximal and distal stumps may differ in relation to the length of the defect. Injury with a segmental gap precludes tension-free, end-to-end coaptation repair. There has been some disagreement regarding whether or not there is an ideal nerve defect that will allow neural regeneration and achieve functional recovery. Nerve injury repairs of short defects, between 0.5 and 5.0 mm, have been producing varying results. Studies of segmental nerve defects have revealed the existence of a critical nerve gap length where the efficacy of conduits starts to decline. These different types of nerve conduits have been used to provide foundation for nerve regeneration to occur in nerve gaps that are less than 3 cm; however, animal models examining nerve regeneration in nerve gaps of 3 cm or more have not shown promising results. Mackinnon and Dellon detailed that the primate peripheral nerve could regenerate across a 3-cm nerve gap when guided appropriately. They extrapolated their findings to humans with nerve gaps of 3 cm or less and demonstrated excellent recovery in 33% of the patients.
Other experiments have suggested that axons should be able to align themselves in response to neurotrophic factors when allowed to grow across a conduit. This finding suggested that conduit repairs might lead to improved functional results, compared with standard end-to-end repair. Different growth factors with different conduit luminal scaffolds such as collagen and laminin have been used. These modifications, however, did not offer substantial benefit over using autografts ; thus, continued investigation is being conducted to find the effective combination of scaffold, cells, and signaling factors that will yield better neural regeneration outcomes.
Degeneration of the Target End Organ
Denervation atrophy of target tissue is another important challenge that is associated with peripheral nerve repair and regeneration. Aside from the long distances that nerves need to traverse to reach their target organs, prolonged target deprivation is found to reduce the ability of motor neurons to regenerate and cause SCs to lose their growth-supportive phenotypes. Wallerian degeneration occurs distal to the injury site, and communication is lost between the nerves and the muscles that they innervate. It has been shown in animal models that after the loss of innervation, the acetylcholine receptors located on the muscle membrane degenerate, as evidenced by decreased density and altered morphology. Furthermore, long-term denervation leads to the disassembly of the motor endplates, causing the acetylcholine receptors to redistribute throughout the muscle fiber. The degradation of motor endplates render the target organ nonviable for the regenerating nerve despite reaching the target. There are findings that suggest that after injury, regrowth into the muscle becomes successful. However, even with successful regeneration, there is still a failure to reestablish motor function, suggesting a possible critical role of the neuromuscular junction. A recent study confirmed that regenerating fibers can reinnervate distal muscles and can reestablish structurally reformed Neuromuscular junction (NMJ) even after several weeks of denervation.
The problem of end-organ atrophy is partially addressed with nerve transfer procedures as there is less time for degeneration with the shorter distance that regeneration must occur; yet, a successful nerve transfer only restores partial muscle function, and preinjury strength is not fully recovered. It is then of great interest to determine if stabilization of the NMJ could potentially reduce end-organ atrophy after nerve injury. Chao et al. provided the first evidence that preservation of the NMJ after traumatic nerve injury improves functional recovery after surgical repair. Matrix metalloproteinase 3 (MMP3) is the major enzyme responsible for the degradation of agrin in denervated muscles which is secreted by terminal SCs. Genetic deletion of MMP3 leads to sustained levels of agrin in denervated muscle endplates, which in turn was able to preserve motor endplate integrity for at least 2 months after nerve degeneration. Taking these into account, these data detail that it is possible to extend the optimal window beyond which destabilization of the motor endplate limits reinnervation. Pharmacological inhibition of MMP3 and local augmentation of neural agrin are a few innovations that could feasibly stabilize the NMJ and diminish end-organ atrophy after nerve injury. Moreover, the canonical Wnt/beta-catenin pathway has been implicated as a potential source of motor endplate instability after long-term denervation. Therefore, targeting the Wnt/beta-catenin pathway may offer therapeutic opportunities for more effective reinnervation of endplates and subsequently improve meaningful functional recovery.
Biologic Augments in Peripheral Nerve Repair
Nerves that are injured do not spontaneously heal and repair themselves. The nerve continuity needs to be reestablished for the nerve to regain its function. The primary goal of nerve repair is to correctly align and approximate severed nerve segments to allow reinnervation of the target organs with hopes of achieving functional recovery. Historically, it was thought best to wait 3 weeks for the completion of the Wallerian degeneration process before nerve repair. However, studies by Fu and Mackinnon detail that immediate repair produced better outcomes. Major prerequisites to nerve repair include a clean wound, viable blood supply, no crush component to the injured nerve, adequate soft tissue coverage, skeletal stability, and minimal tension on the nerve repair. The current principle for a successful nerve repair is the coaptation of nerve tissue without tension and is uniformly accepted as the single most important technical factor in peripheral nerve repair. Treatment of nerve injury continues to be a challenge as early studies detailed poor functional results after surgical repair. But with the increasing understanding of the nerve anatomy and physiology, accompanied by advances of microsurgical technology including utilization of biological augments and refinement of surgical techniques, outcomes of nerve repair have started to be promising. The challenge being posted to reconstructive surgeons today is the repair and reconstruction of long nerve gaps. The following section gives a review of the different tools that are available in our current armamentarium for improving and enhancing peripheral nerve regeneration.
Autogenous Biological Conduits
Autogenous nerve grafting provides a guided pathway composed of lamina scaffold and SCs to allow nerve regeneration. Disadvantages of autogenous nerve graft include the associated patient morbidity and poor functional outcomes, with reported postoperative strength most often only reaching grade 3. Furthermore, autologous donors are usually smaller in caliber, shorter in length, and less abundant. In 1891, Bügner reported successful nerve regeneration with the use of human arterial grafts to bridge sciatic nerve gaps in dogs. The use of artery as a conduit has not been implemented clinically, most likely due to lack of suitable donor vessels.
Unlike arteries, vein autografts are more available and easier to harvest. Wrede, in 1909, was the first to introduce the use of vein grafts for repairing nerve defects. In his study, he reported successful human median nerve repair using a 45-mm long vein tube. Multiple animal studies have also confirmed successful nerve defect repair using vein grafts. Chui et al. reported histologic and electrophysiological evidence of nerve regeneration in 1-cm sciatic nerve gaps in rats. Rice et al. used femoral vein grafts to repair sciatic nerve defects in rats and reported axonal penetration into the distal sciatic nerve segment, concluding that vein grafts are suitable conduits for nerve repair. Seumatsu et al. evaluated nerve repair by vein grafting and by nerve grafting in 1-cm rat sciatic nerve defects and concluded that there was no difference in myoelectrical response between the two groups at 6 months. Vein grafts have also been studied clinically. In a prospective study evaluating patients who underwent nerve repair with autogenous vein grafts and nerve grafting for symptomatic neuroma, Chiu and Strauch reported that even though superior results were found in patients in the nerve graft group, patients in the vein graft group had successful return of two-point discrimination. In 1989, Walton et al. reviewed results of 22 patients with digital nerve repairs using autogenous vein grafts and reported good results for acute digital nerve repairs but poor results with delayed digital nerve repairs. More recently, Rinker and Liau demonstrated equal sensory recovery after digital nerve repair (mean nerve gap of 10 mm) with autogenous vein grafts to repair with collagen conduit, with vein grafts yielding fewer complications. Overall, the use of vein grafts has shown potential for nerve repair.
The main concern with the use of vein grafts is the possibility of lumen collapse, which can then impede nerve regeneration. This remained a concern even though Tseng et al. demonstrated that the vein graft remains patent during the process of nerve regeneration. Efforts to limit the risk of vein collapse led to the filling of vein grafts with muscle or nerve tissue. These intraluminal additives have benefits of supplying extracellular matrix and neurotrophic factors that facilitate nerve regeneration across defects by promoting SC proliferation, migration, and axonal growth cone guidance.
Veins that are interposed with neuronal tissue have shown to produce meaningful recovery results in ulnar, median, superficial radial, and digital defects of 2.5–4.5 cm.
The use of skeletal muscle for nerve repair was prompted by the early observation that skeletal muscle fibers have a basal lamina oriented in a longitudinal fashion resembling endoneurial tubes of degenerating nerves. Potential skeletal muscle fiber donor sites are numerous; however, there is a risk that nerve fibers can grow out of the muscle tissue during nerve regeneration. Both fresh and denatured muscles have been used in studies. Glasby et al. used degenerated muscle grafts to repair a 30-mm nerve gap in adult marmosets and reported successful regeneration. Pereira et al. used denatured muscle grafts for reconstruction of digital nerve defects between 15 and 28 mm and reported better results than nerve grafting. However, not every study reported positive results. Raganovic et al. reported worse quality of recovery in patients with radial nerve defects repaired with denatured muscle grafts compared with outcomes of nerve graft repair.
Another biologic conduit that has been studied for nerve repair is the tendon autograft because of its collagen content and ECM components. Using a rat model, Brandt et al. suggested that tendon grafts can be used as nerve conduits to bridge 10- and 15-mm gap defects. Even though the aforementioned studies show tendons as a feasible neural gap conduit because of the lack of clinical studies, it is still uncertain if tendon autografts are useful in human nerve repair.
Nonautogenous Biological Conduits
Nerve allografts have been studied as a potential alternative for nerve repair. Nerve allografts provide a readily available tissue source for nerve reconstruction. The first clinical results from the use of nerve allografts dated back in 1885 reported poor clinical outcomes. Multiple early studies on nerve allografts suggested that the likely cause of clinical failure is immune rejection, which can lead to scarring and fibrosis and eventually act as a mechanical barrier for regenerating fibers. The advent of host immunosuppression allowed for nerve allografts to be a possible reconstruction alternative for nerve repair. Techniques such as administration of concurrent immunosuppressants and pretreatment with radiation and lyophilization have been used to combat the problem of immunogenicity. The allograft is believed to serve as a temporary scaffold for regenerating host nerve fibers. As regeneration proceeds, the donor determinants within the allograft are slowly replaced by host components. Mackinnon et al. applied the concept of allografting with immunosuppression clinically in patients with large peripheral nerve gaps. In this study, return of motor and sensory function was observed, and only 1 patient experienced rejection secondary to subtherapeutic immunosuppression.
Decellularized nerve allografts (DCAs) were developed in efforts to completely eliminate the need for immunosuppression as it imposes harmful side effects. In DCAs, human nervous tissues are processed to render the grafts nonimmunogenic, hence precluding the need for immune modulation but at the same time retaining the physical macrostructure that facilitates nerve regeneration. In DCAs, host SCs are considered the driving force for regeneration as they migrate from neighboring nerve stumps and create the neurosupportive environment to encourage axonal regeneration. While processed nerve allografts are acellular, they are able to revascularize and repopulate with host cells, thus providing an environment that is conducive for regeneration. Methods of nerve decellularization include freeze thawing, irradiation, detergent processing, enzyme digestion, and cold preservation. AxoGen is currently the only commercially available allograft that is approved by the Food and Drug Administration (FDA) for clinical use. The propriety preparation of AxoGen allografts is a combination of detergent processing, enzyme digestion to remove axonal growth inhibition by chondroitin sulfate proteoglycans, and gamma irradiation. The decellularization process alters the molecular and structural properties of the nerve, and the specific method of preparing the acellular nerve allografts can actually affect the overall quality of regeneration. Moore and colleagues experimented with rat nerves that were processed using different methods of decellularization to bridge a 14-mm nerve defect in an animal model. In their study, they showed that allografts processed with detergent had similar performance to autografts, whereas allografts prepared by either cold preservation or AxoGen processing showed inferior nerve regeneration in comparison to autografts. It was theorized that the detergent processing allowed retention of growth factors released from the ECM. Yet, Whitlock et al., via a rat sciatic model, showed that regeneration across gaps of 14 and 28 mm using AxoGen allografts was inferior to autografts. Guisti et al. evaluated return of motor function in rats with 10-mm sciatic nerve defects treated with autografts, DCAs, and collagen conduits. They concluded that processed allografts were overall inferior to autografts but resulted in better motor function than those that were treated with collagen conduits.
There have also been studies looking at the efficacy of nerve allografts in humans. In 2009, Karabekmez and colleagues reported the first study to examine the short-term clinical nerve function recovery after nerve repair with AxoGen nerve allograft. In this study, they concluded that DCAs are capable of adequate sensory repair in nerve defects up to 30 mm without infection or rejection. Brooks et al. published results from the first multicenter trial on the Avance (AxoGen) processed allograft. The study was based on a total of 76 peripheral nerve defect injuries (5–50 mm) which included 49 sensory, 18 mixed, and 9 motor nerves. They reported 87% meaningful sensory and motor recovery based on the Mackinnon grading system. Guo et al. evaluated digital nerve repair using DCAs in 5 patients with an average defect of 23 mm. They reported S3+ or better sensation in all subjects and an average two-point discrimination of 6 mm at 13-month follow-up. In 2015, Rinker and colleagues reported on 37 short-gap sensory repairs using DCAs after which 92% of subjects showed meaningful recovery. Cho et al. demonstrated recovery of M3 or better motor function in 75% of median nerve repairs and 66% of ulnar nerve repairs. The use of DCA in repair of long nerve gap was also documented. Fleming et al. confirm return of motor and sensory function in a patient who underwent DCA ulnar nerve repair for a 70-mm nerve defect. Multiple studies have shown that decellularized nerve allografts seem to provide promising results; however, not all outcomes of studies have shown such promise. Cho et al. reported that 2 of their subjects reported no functional recovery. Of note, one injury is a 491-day-old median nerve injury, and the other was a high-energy blast injury to the ulnar nerve. Berrocal and colleagues reported a case of failure of DCA repair after a high-energy fracture of the distal ulna, highlighting the importance of nerve diameter and length of defect in successful repair.
Consistently, attempts of functional recovery over larger nerve gaps >30 mm have been more difficult to achieve. In 2013, using an animal model, a study looked at SC senescence in acellular nerve allografts during nerve repair. Interestingly, they observed high evidence of cellular senescence in DNAs with gaps >60 mm. Clinical length limitations in DCAs have been debated. AxoGen’s Avance is supplied in lengths between 15 and 70 mm, with internal diameters between 1 and 5 mm.
Critical analysis of data presented previously revealed that the best results were in digital nerves, i.e., pure sensory nerves with limited gap. Although some authors concluded that the processed nerve allografts could be effectively used in nerve gaps of 5–50 cm in length, this claim has not been widely accepted in clinical practice. The upper limit for clinical use of DCAs is not yet fully established and is most often used when the magnitude of nerve injuries exceed the capacity of donor autografts.
Synthetic Conduits
Synthetic conduits work by encasing the distal and proximal ends of the nerve within a tube, allowing for macroalignment for the nerve. Fibrin matrix then forms between the nerve ends that can support cellular migration between the nerve endings. As cells start to invade the cable, band of Büngner form within the disorganized matrix to allow for neurite outgrowth. This mechanism depends on the volumetric output from the nerve stumps. The earliest success with synthetic conduits was observed back in 1898 by Merle et al., who bridged nerve defects with silicone polymer nerve guides. Lundborg and colleagues then published several reports illustrating the feasibility of silicone conduits for nerve repair and reconstruction. Moreover, a study by Braga-Silva reported effective late repair with silicone tubes in peripheral nerve injuries with gaps of up to 30 mm. Despite the early promising results, the nonresorbable nature of the silicone tube caused permanent fibrotic encapsulation of the implant with subsequent chronic nerve compression. In fact, these tubes have been used by many investigators to create Chronic Nerve Compression (CNC) injuries in animal models. This critical limitation led to the development of absorbable synthetic conduits that will allow diffusion of oxygen and micronutrients across the outer walls and into the matrix. There are 3 types of synthetic conduits that are FDA approved, each made from different biomaterials: (1) polyglycolic acid (PGA), (2) collagen type I, and (3) caprolactone. The use of these conduits is currently limited to defects of 3 cm or smaller; however, with the advent of bioengineering, there is a potential for these conduits to be an option for larger nerve defects. Of the FDA-approved nerve conduits, the collagen-based NeuraGen and the PGA-based NeuroTube conduits have the most encouraging clinical data to date.
Polyglycolic Acid
PGA is a commonly used suture material and was the first material used to construct nerve conduits when the limitations of silicone tubes were observed. It has excellent mechanical properties and is rapidly degraded into lactic acid. PGA conduits have yielded positive outcomes in both sensory and motor recovery in segmental nerve defects. Animal studies showed early support for PGA tubes as good alternatives to nerve grafts. Dellon et al. described histologic and electrophysiologic evidence of regeneration that is achieved after PGA conduit repair of a 30-mm ulnar nerve gap in monkeys and concluded that there is no significant difference when compared with repair with sural nerve graft. Matsumoto et al. reported successful regeneration across an 80-mm nerve gap in dog peroneal nerve with the use of a PGA conduit. PGA conduits also were used in human nerve reconstruction. Mackinnon and Dellon reported a case series of 15 secondary reconstructions of digital nerve defects 30 mm or smaller and reported 86% meaning recovery. Weber and colleagues reported on the first randomized prospective multicenter evaluation of the first commercially available PGA conduit, NeuroTube, for digital nerve repair. NeuroTube was approved by the FDA in 1999 for human use in the United States. The study concluded that in nerve defects of less than 40 mm, NeuroTube provided significantly better return of sensory function than direct suture repair. Efforts to extend the use of PGA to larger nerve defects and motor nerves were undertaken by Rosson et al., who bridged median nerve defects (15–40 mm) and reported meaningful recovery in all patients. Subsequent studies comparing PGA to other methods of nerve repair found PGA to perform equally, if not better. For example, Battison and colleagues compared PGA nerve repair to nerve repair with autogenous vein grafts filled with muscle. In this series of 17 patients with 19 digital nerve injuries repaired using NeuroTube conduits across 10–40 mm nerve gaps showed positive results and no differences in functional recovery between the 2 cohorts. In 2011, Rinker et al. compared PGA conduits and autogenous vein grafts for both short (<10 mm) and long (>10 mm) digital nerve defects and found no difference in meaningful recovery between the two groups. A documented disadvantage of the NeuroTube is the extrusion of the conduit even in healthy-looking tissues. Weber et al. reported 46 cases of PGA conduit implantation, 3 of which got extruded. Duncan and colleagues reported on a patient with a radial digital nerve that was bridged with PGA conduit, who, on postoperative week 4, was found to have extrusion of the conduit through the wound. They mentioned that the cause for this complication was not very clear, but the properties of the PGA conduit and the patient’s own immunological properties are some factors to consider.
Collagen Conduits
Collagen conduits are made from purified type 1 collagen derived from bovine flexor tendons. Collagen is the major component of the ECM and is believed to facilitate adhesion and enhance cell proliferation. The utilization of collagen conduits in nerve reconstruction has been demonstrated both in animal and human studies. Archibald et al. demonstrated the feasibility of collagen nerve guides in regeneration of a 5-mm nerve gap in nonhuman primates. Using rat models, Keilhoff et al. tested collagen type I/III tubes as potential nerve guides and reported that collagen can serve as template to ensure nerve regeneration through nerve defects.
NeuraGen was commercially available in 2001 and has been examined by numerous investigators. Clinical studies evaluating the efficacy of collagen conduits have demonstrated favorable outcomes in defects of 20 mm or less. Ashley et al. used NeuroGen as graft material in five patients with obstetrical brachial plexus palsy. Collagen tubes were used to repair nerve gaps smaller than 20 mm. They reported that four out of the five patients made good functional recovery, being able to dress and feed themselves at 2-year follow-up. In a retrospective study, Bushnell et al. followed up 12 patients with repair of digital injury using NeuroGen bovine collagen nerve tube and reported effective restoration of digital nerve function in the early postoperative period. Lohmeyer and colleagues found that collagen conduits provided consistent functional recovery in nerve gaps that are less than 15 mm. No functional recovery was seen in gaps that are greater than 15 mm. Wangensteen et al., in a retrospective review, reported an overall 35% recovery of quantitative nerve function in nerve defects of 2.5–20 mm and 31% going on revision surgery. In 2011, Taras et al. reported a 73% meaningful recovery in 5–15 mm digital nerve lacerations repaired with NeuroGen.
Several investigators have studied collagen conduit repair in comparison to other methods of nerve repair. In a rat model comparing NeuraGen to Avance (AxoGen allograft), NeuroGen produced inferior results in rat model across 14- and 28-mm gaps. Boeckstyns et al. evaluated and compared repair of acute lacerations of mixed sensory-motor nerves in 43 patients using collagen tube versus traditional neurorrhaphy. The study concluded that the use of collagen conduit produces sensory and motor functional recovery that is equivalent to direct suture in nerve gaps that were 6 mm or less.
There are documented studies that show failed collagen conduits in mixed nerve repair and digital nerve repairs. Liodaki and colleagues reported cases of poor regeneration in 4 patients with unsuccessful implantation of NeuraGen conduit. Evidence of scarring and fibrosis was observed with the conduit and the surrounding tissue bed. Moreover, histologic assessment found neuromas and evidence of foreign body reaction. Other collagen conduits are available in the market; however, there are limited clinical or preclinical data available for NeuroWrap, NeuroFlex, NeuroMatrix, and NeuroMend. As such, it is challenging to draw any conclusions about their clinical utility.
Poly D, L Lactide-co-epsilon-caprolactone Conduits (PCL)
PCL nerve guides are the most recent FDA-approved synthetic conduits. These conduits have the advantage of being transparent in nature allowing good visualization of nerve stumps within the chamber. The PCL material degrades into small particles over a period of time until it is fully resorbed. Its crystalline structure remains impermeable to fluid longer than PGA and collagen conduits. The material itself, however, is very rigid, which renders PCL conduits more difficult to handle in the clinical setting. Efforts to assess the efficacy of PCL conduits for nerve repair have yielded mixed outcomes, and the use of NeuroLac in the clinical setting has been less encouraging. Preclinical data in rat models with a 10-mm sciatic nerve defect using NeuroLac suggested favorable motor recovery comparable to autografts. Bertleff et al. evaluated digital nerve repair of a 20-mm or less nerve gap in 21 nerve lesions. They concluded that recovery results in the PCL group are comparable to those obtained after end-to-end repair. Studies by Meek et al. on digital nerve repair in the foot and Hernandez Cortez et al. on a digital nerve gap in the thumb demonstrated no meaningful recovery when PCL conduits were used. Hernandez Cortez reported that failure of the implant was accompanied by histological evidence of biomaterial fragments and foreign body reactions. Moreover, Chiriac et al., in 2012, analyzed a series of 28 nerve repairs of the upper extremity with PCL conduits and reported only a 25% meaningful recovery with complications such as neuroma formation and fistularization of the conduit into the joint. With conflicting results presented previously, the efficacy of PCL conduits for clinical use remains largely unclear.
Exogenous Agents
The addition of exogenous neurotrophic factors in peripheral nerve injury has garnered attention in the past few years. Presently, there are no clinically available pharmacological treatments for nerve injury; however, there are several small molecules, peptides, hormones, and growth factors that have been studied and have been suggested as potential candidates to improve nerve regeneration. The addition of factors such as NGF, BDNF, and Human ciliary neurotrophic factor (CTNF) in conduits used for nerve repair shows to enhance axonal outgrowth and improve remyelination and speed of axonal regeneration. Derby et al. reported positive effects on neuronal regeneration in rat sciatic models of 10- and 15-mm defects when NGF was added to silicone chambers. In 1999, Ho and colleagues evaluated if BNDF and CNTF in collagen tubules can successfully achieve functional recovery in rat sciatic nerve defect model. They concluded that BDNF and CTNF can be covalently linked to collagen tubules to allow regeneration and that cotreatment with both BDNF and CTNF produced the best functional recovery in their model. Another animal model reported that rat tibial nerves (of 8-mm gap) reconstructed with fibroblast growth factor within a silicone conduit had 30% more regenerating axons than autografts. Walter et al. evaluated the effects of recombinant FGF on conduit bridging a 15-mm nerve gap. They reported functional motor recovery and increased muscle action potential in the Fibroblast growth factor (FGF) group compared to the control group. Wang et al. also experimented on rat sciatic nerve model and documented enhanced peripheral nerve regeneration when using PCL tubes containing FGF to repair 15-mm nerve gaps. GDNF is another neurotrophic factor secreted by SCs after nerve injury which is known to improve neuronal survival and outgrowth. Fine and colleagues used a rat model and demonstrated enhanced motor and sensory neuronal regeneration across a 15-mm synthetic nerve tube with NGF and GDNF inclusion. Lee et al. used heparin-containing delivery system to slow the diffusion of NGF from a fibrin matrix in a conduit bridging a 13-mm gap in rats. The study revealed a dose-dependent effect of NGF on nerve regeneration and that the total number of nerve fibers was similar to that of isografts. Marquardt et al. used scaffolding containing GDNF and tetracycline-inducible GDNF overexpressing SCs to repair a 30-mm defect in a rat model. In this study, GDNF was delivered in a well-controlled manner both spatially and temporally. Results showed enhanced axonal regeneration which in turn resulted in increased gastrocnemius and tibialis anterior muscle mass. Glial growth factor is a trophic factor specific for SCs and plays an important role in the interaction between SCs and neurons and is theorized to increase SC motility and proliferation. Using a rabbit common peroneal nerve transection model, Mohanna et al. demonstrated a progressive increase in SC number and axonal regeneration when glial growth factor is applied into polyhydroxybutyrate conduits bridging nerve gaps of 20–40 mm. Vascular endothelial growth factor (VEGF), Leukemia inhibitory factor (LIF), and PDGF are other examples of neurotrophic factors that have demonstrated some efficacy in peripheral nerve repair but are used less.
Additional agents have been investigated to help improve outcomes in peripheral nerve repair. Betamethasone was studied to assess its effect on peripheral nerve regeneration and functional recovery after sciatic nerve transection and repair of a 10-mm defect in rat models. Functional study confirmed faster recovery of regenerated axons in the betamethasone group. Thyroid hormone (T3) was also investigated as a possible agent to enhance regeneration. In their rat animal study, Papakostas and colleagues observed the effects of T3 hormone on peripheral nerve regeneration as it was injected into silicone tubes to bridge nerve gaps. They reported accelerated return of sensory function without significant effect on motor nerve recovery. Vitamin E and pyrroloquinoline (PQQ) were studied using a rat sciatic nerve transection model and it was found that the combination of the two provides better functional recovery of nerve regeneration than Vitamin E or PQQ solely.
More recently, local rapamycin has been studied in a model of rat sciatic nerve defect of 15 mm. It was found that rapamycin is able to reduce secondary nerve injury and promote regeneration of peripheral nerve. Around the same year, Shahraki et al. reported on the effects of tacrolimus (FK506) on nerve regeneration in a rat sciatic nerve model. In this study, they concluded that the administration of FK506 could accelerate functional recovery of the sciatic nerve after nerve allografting. Despite positive results in animal studies of nerve repair, these agents have not been widely used due to mixed and limited demonstrable efficacy in humans as well as the possible adverse effects of the drugs. There are a few human studies however that included tacrolimus as an essential part of the immunosuppression protocol. FK506 has been reported as an agent used for immunosuppression in cases of human upper extremity transplantation. Martin et al. in 2005 demonstrated successful autoreplantation of a proximal arm with 1-year treatment of oral tacrolimus. Authors reported electromyographical improvements in the ulnar and median nerve distribution. In another study, Schuind et al. reported return of intrinsic muscle function as well as a two-point discrimination of 6 mm at the thumb and index finger after a hand transplant with tacrolimus as part of their immunosuppression protocol. In 2011, 9 years after hand transplant, authors report that the patient is able to go back to work. Focusing more on nerve repair, Phan and Schuind looked to investigate the effects of FK506 on axonal regeneration after nerve suture or autograft repair. In their study which included 5 patients with nerve transection, they concluded that FK506 did not demonstrate evidence that it enhances nerve regeneration, stating that the timing and duration of tacrolimus treatment may not have been optimal. The aforementioned human studies mentioned no major side effects of tacrolimus during the duration of treatment.
In general, these factors mentioned previously are either injected directly into nerve conduits or embedded within a hydrogel and can be used on their own or in combination with each other. For the future, continued research should focus on the importance of neurotrophic factor dose response and understand how possible combinations of these factors can be used to create optimal scaffold designs for nerve regeneration.
SC Transplantation
SCs, as discussed previously, play an important role in mediating nerve regeneration. Thus, SCs seem to hold the most promise in nerve restoration. SCs have been seeded onto synthetic conduits to enrich the regenerative environment and improve regeneration during nerve repair and reconstruction. In most experimental studies published so far, significant improvement in morphological and functional recovery have been observed. Strauch and colleagues reported excellent nerve regeneration through a 60-mm venous nerve conduits seeded with autologous SCs in their animal model. Furthermore, another animal study by Hoben et al. reported the addition of SCs in acellular nerve allografts improves peripheral nerve regeneration in 20-mm nerve defects. One of the initial concerns with using SCs for nerve repair is that the process of culturing SCs can take a long time. There are now novel techniques available that address this challenge, yielding SC cultures in a shorter period of time (about 2 weeks).
An SC–stem cell cocultured system has also been studied in the animal models of 15-mm nerve defect. In this study, Dai et al. suggested that when incorporated to nerve conduits, sciatic nerve repair with the SC–stem cell coculture resulted in functional recovery, as evaluated by the walking track, functional gait, nerve conduction velocity (NCV), and histological analysis. More recently, Xu and his colleagues also described an SC–stem cell coculture system in their animal model used to seed scaffolds to bridge 10-mm nerve defect in rats and reported regenerative results that are equal to that of autologous nerve grafts but superior to those repaired with plain nerve scaffold. Although there are many studies reporting the benefits of SCs in animal models, clinical trials have not been reported to date. The University of Stanford investigated use of cultured SCs for peripheral nerve injuries of short and long gaps; however, no clinical data from the study have been documented in the literature.
More recently, Levi et al. published the first human experience of using autologous SCs grown from sciatic nerve and sural nerve graft. SCs were seeded onto sural nerve graft to repair sciatic nerve defect of 7.5 cm. After repair, the patient had evidence of sensory and motor recovery distal to the repair. This seminal work supports the possibility of cellular therapy to improve functional neural recovery. We predict that the recent advances in stem cell biology will continue to offer clinical use of SC-enriched conduits because there is an overwhelming evidence that SCs are able to be derived from stem cells.
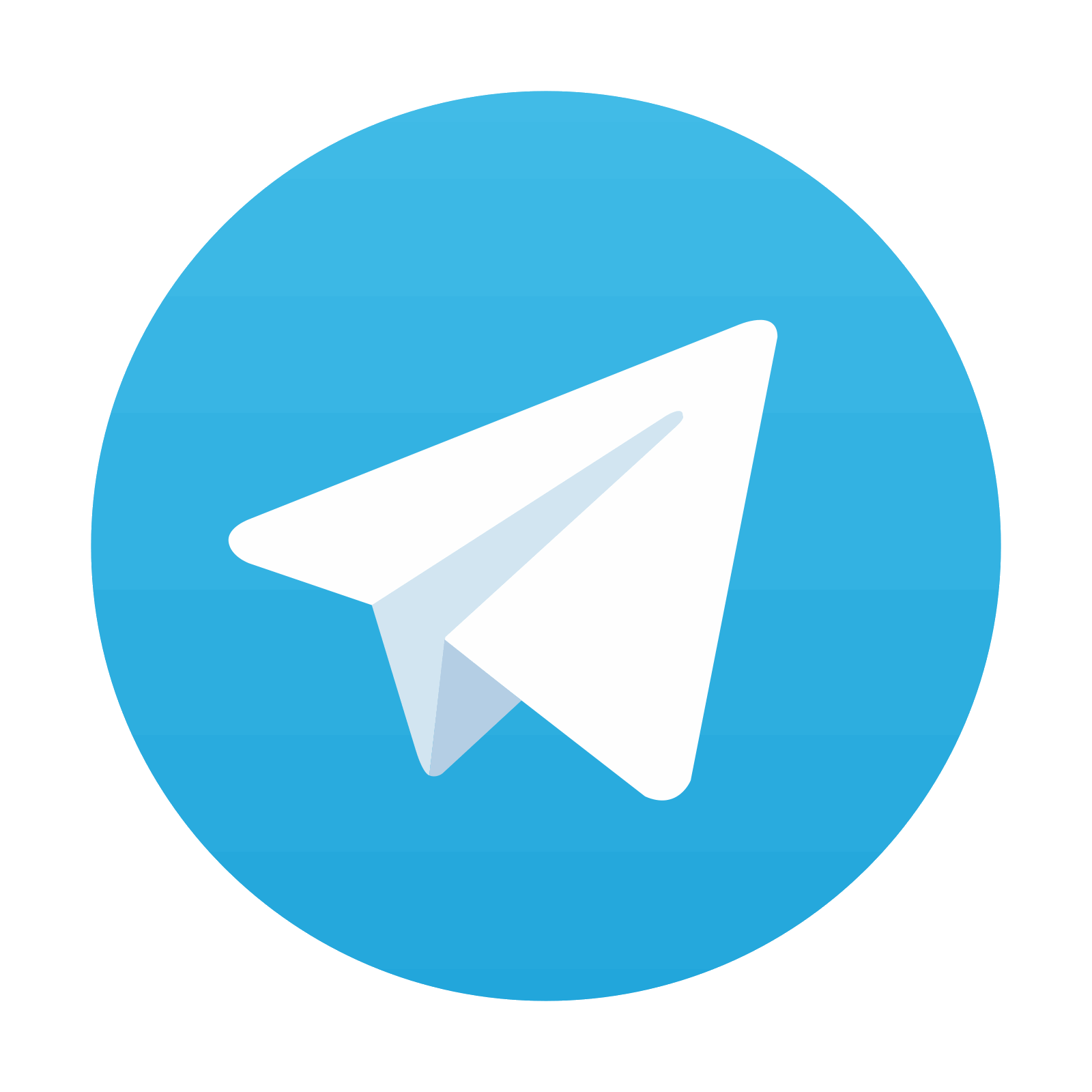
Stay updated, free articles. Join our Telegram channel
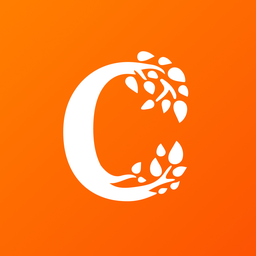
Full access? Get Clinical Tree
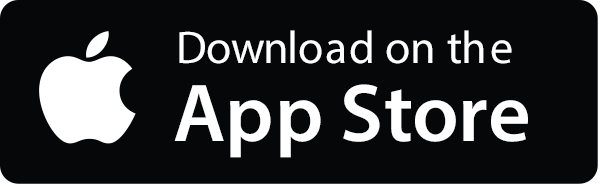
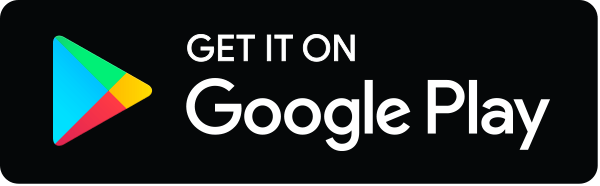