Basic Science of Polyethylene
Ebru Oral
Orhun Muratoglu
Introduction
Contemporary total joint arthroplasty evolved from technology developed by McKee (in 1951) and Charnley (in 1958) for the artificial hip (1,2). The most widely accepted implant configuration for joint arthroplasty since its inception comprises a metal-on-polymer articulating component pairing, where the polymeric bearing surface is fabricated from ultrahigh–molecular-weight polyethylene (UHMWPE). Other bearing couples such as metal-on-metal (MoM) or ceramic-on-ceramic total joint replacements have been routinely used, but metal-on-UHMWPE articulations are the most common for degenerative joint disorders (3). The number of joint implants are estimated to be over 1 million total joint replacements annually in the United States alone (4,5), making UHMWPE one of the most widely used biomaterials in the world.
Although the quality of life for most arthritis patients is significantly increased as a result of the surgery (6), total joint arthroplasty revisions are increasing steadily with an estimated 200,000 patients undergoing revisions each year, the cost of which is approximately $6 billion (5,7,8). In hips, the common causes for revision are dislocation (23%), infection (15%), and mechanical loosening (20%) (9). Whereas infection and dislocation remain early-stage complications, osteolysis and related instability that occur at the later stages have been linked mainly to the wear particles that result from the wear of the articulating surfaces, especially UHMWPE (10,11). Radiation cross-linking of the polymer, accompanied by thermal treatments to improve oxidative stability, has successfully decreased wear and the incidence of osteolysis in the first decade of service. For further improvement of fatigue and oxidation resistance, several alternative antioxidant-stabilized UHMWPE bearing surfaces have been introduced.
The current driving force behind new developments in UHMWPE as a bearing surface is not only reducing revision surgeries in the current population, but also addressing the needs of the growing population of younger patients. From 1990 to 2002, the percentage of patients aged 45 to 64 undergoing primary total hip replacement increased from 28% to 42% (4). Metal-on-metal implants were increasingly favored for younger patients (8) due to the availability of larger head sizes with minimal concern about oxidative degradation, dislocation, and fatigue failure but with caution on the long-term effects of metallic debris and metal ions (12). Unfortunately, significant complications in the periprosthetic tissue of MoM implants (13,14,15,16) have prompted the Medicines and Healthcare Products Regulatory Agency of the United Kingdom to issue an alert regarding these implants (17) and have promptly and drastically reduced their use (15,18). Therefore, the demands on the performance and longevity of metal-on-UHMWPE implants have accordingly increased to include a larger and more demanding population of patients.
The present chapter provides a review of the structure, morphology, and properties of UHMWPE as related to its processing and use as a bearing surface for total joint replacement. The first part summarizes the effects of processing on the structure and relevant properties of UHMWPE, the second part relates information on currently available cross-linked UHMWPEs with and without antioxidants and the review ends with newly introduced and pending formulations of cross-linked UHMWPEs.
Structure and Properties of Uhmwpe Resin Powder
Polyethylene is a polymer comprising –CH2– monomeric entities. Although its structure is simpler than most polymers, it can have a variety of properties depending on its molecular weight and branch structure. Polyethylene can be in the form of linear low-density polyethylene (LLDPE), very low-density polyethylene (VLDPE), low-density polyethylene (LDPE), high-density polyethylene (HDPE), and UHMWPE. The nomenclature of UHMWPE has changed considerably in the past four decades beginning in the early 1960s as a form of HDPE (19) and changing as it became possible to produce even higher–molecular-weight polyethylenes. Thus, Charnley’s reference to the UHMWPE he used as HDPE (20) was historically correct. Today, a linear polyethylene with a density greater than 0.940 g/cm3 and a molecular weight typically below 200,000 g/mol is defined as HDPE, and UHMWPE is defined by ASTM D 4020 as a linear polyethylene with an average molecular weight greater than 3.1 million g/mol (21) and greater than 1 million g/mol by ISO 11542. The two currently available UHMWPE resins are designated GUR1020 and GUR1050 and have molecular weights of approximately 3.5 and 6 million g/mol, respectively (22). Until the disassembly of
their production line in 2002, two other resins—1900 and 1900 H, which had a molecular weight in the range of 2 to 4 million g/mol—were supplied by Hercules Powder Company and Montell Polyolefins (Wilmington, DE, USA). The discussion of these resins from a historical perspective of structure–property relationships will later follow.
their production line in 2002, two other resins—1900 and 1900 H, which had a molecular weight in the range of 2 to 4 million g/mol—were supplied by Hercules Powder Company and Montell Polyolefins (Wilmington, DE, USA). The discussion of these resins from a historical perspective of structure–property relationships will later follow.
At the nanometer scale, UHMWPE is a semicrystalline polymer with its crystalline domains embedded within an amorphous matrix (Fig. 14.1). The crystalline phase of UHMWPE consists of lamellae (folded rows of carbon; Fig. 14.1), typically 10 to 50 nm in thickness and on the order of 10 to 50 μm in length. There is a distribution of lamellar sizes within the polymer with the surrounding amorphous phase consisting of randomly oriented and entangled polymer chains traversed by tie molecules that interconnect lamellae and provide resistance to mechanical deformation. Thus, UHMWPE is a complex, viscoplastic composite which can evolve over time in response to its mechanical, chemical, and thermal history.
The intrinsic viscosity of UHMWPE, which increases nonlinearly with increasing molecular weight (23), is a determinant of the bulk impact strength and abrasive wear resistance of the polymer after conversion to bulk form. The maximum impact strength was found to be at a molecular weight range of 2.4 to 3.3 million g/mol (19,22). As the intrinsic viscosity increases, abrasive wear resistance increases reaching a plateau at approximately a molecular weight of 3.3 million g/mol. Thus, the wear resistance of UHMWPE is the highest among polyethylenes and in vitro wear studies using pin-on-disc (POD) apparatus, the wear of UHMWPE consolidated by compression molding without further processing varied between 7 and 10 mg/million-cycles of testing (24,25).
In addition to the molecular weight, the macroscopic material properties of different UHMWPE resins may also be dependent on the average resin particle size, the size distribution, and morphology of the resin particles (26), the differences of which have been attributed to the use of different catalysts and manufacturing processes. The aforementioned GUR resins have a mean particle size of approximately 140 μm, whereas the mean particle size of the 1900 resin was nearly 300 μm. It was found that the particle morphology of the GUR resins comprise a fine network of submicron-sized fibrils which interconnect characteristic microscopic spheroids (19). Depending upon the processing conditions, the 1900 also exhibited spherulitic crystalline morphology (27), whereas spherulites are not observed in GUR resins, which have a lamellar crystalline morphology (28). Because of the various crystalline morphologies possible in different types of resins, the tendency of each for further chemical interactions such as cross-linking or oxidation may be different as well. For instance, the 1900 resin has had a relatively high long-term success; implants which had been fabricated by direct compression molding of this resin showed higher survival rates due to their resistance to oxidation compared with implants fabricated from the conventional GUR resin of similar molecular weight (29).
In addition to the properties of the resin itself, consolidation of the resin powder into large-scale forms which can be used as or machined into implants also contributes to the properties of UHMWPE as a clinical bearing surface. The resin powder, historically provided by only a handful of chemical manufacturers, is converted to consolidated forms of stock either by bulk converters or by medical implant manufacturers. Because the melt viscosity of UHMWPE is very low, it is not amenable to typical processing methods such as injection molding. Consolidation is done either by compression molding or a special extrusion process called ram extrusion (30). Implants can then be machined from consolidated stock or resin powder can be “direct compression molded” into the final implant shape. In addition to direct compression molding and machining of converted stock material, there exists a hybrid UHMWPE manufacturing process used by Biomet known as HIPing (Hot Isostatic Pressing) (31). The multistep process begins with the manufacture of a cylindrical compact through cold isostatic pressing which expels most of the air. Subsequently, the compacted rods are sintered in a hot isostatic pressure furnace in a low-oxygen pouch to prevent degradation. The resulting rod stock is essentially isotropic due to the hydrostatic sintering process and may be considered to be a compression-molded form of the resin. The mechanical and wear properties of UHMWPE may be significantly affected by the manufacturing processes, such as the temperature and pressure cycles during molding and, as such, these processes are proprietary.
The mechanical properties of UHMWPE are strongly related to its crystalline content and structure and the amount of interaction between its crystalline and amorphous phases.
For example, the mechanical properties of consolidated UHMWPE vary as a function of the cooling rate during consolidation (32), which affects the crystallization kinetics and may lead to lower crystallinity at higher cooling rates. For example, a slow cooling rate from 142°C under nitrogen produced a compact that had a modulus of 475 MPa and a yield strength of 21.7 MPa, whereas water quenching from 200°C produced a compact with a modulus of 213 MPa and a yield strength of 13.8 MPa. In contrast, raising the temperature and pressure during melting to above approximately 210°C and 290 MPa results in a phase change in UHMWPE where the crystals exhibit hexagonal morphology and the lamellar formation is thought to be less inhibited (33,34). In this “extended chain crystal” morphology (Fig. 14.1A), the crystal size and the crystalline content increase, resulting in substantially improved mechanical strength up to a yield strength of 27.6 MPa and an ultimate tensile strength of 65 MPa (25,33,34).
For example, the mechanical properties of consolidated UHMWPE vary as a function of the cooling rate during consolidation (32), which affects the crystallization kinetics and may lead to lower crystallinity at higher cooling rates. For example, a slow cooling rate from 142°C under nitrogen produced a compact that had a modulus of 475 MPa and a yield strength of 21.7 MPa, whereas water quenching from 200°C produced a compact with a modulus of 213 MPa and a yield strength of 13.8 MPa. In contrast, raising the temperature and pressure during melting to above approximately 210°C and 290 MPa results in a phase change in UHMWPE where the crystals exhibit hexagonal morphology and the lamellar formation is thought to be less inhibited (33,34). In this “extended chain crystal” morphology (Fig. 14.1A), the crystal size and the crystalline content increase, resulting in substantially improved mechanical strength up to a yield strength of 27.6 MPa and an ultimate tensile strength of 65 MPa (25,33,34).
The consolidation process requires effective fusing of the polymer resin particles, which requires the long polymer chains at the granule boundaries to diffuse effectively into the neighboring granule. Dynamic thermal analysis of entangled UHMWPE (molecular weight, 3.6 × 106 g/mol) showed a terminal relaxation time of 15 hours at 180°C (35), much longer than the conventional processing time (usually less than 1 hour at 190° to 250°C). Thus, fusion defects can occur. Although type 1 fusion defects (incomplete interparticle voids) can be removed by properly controlling the consolidation conditions, type 2 fusion defects (incomplete interparticle cohesion by self-diffusion) are common in UHMWPE, even in well-consolidated stock. One method by which such defects can be decreased may be prolonged exposure to high temperatures (∼300°C), which has been shown to significantly enhance impact toughness (36). A highly impact and oxidation-resistant cross-linked UHMWPE processed using this method and stabilized by the antioxidant vitamin E has been recently developed and will be discussed in the last section.
![]() Figure 14.2. A selection of conventional, gamma-sterilized retrievals showing excessive wear and delamination with time. (A) 8 years, (B) 13 years, (C) 17 years, and (D) 24 years. |
Understanding Oxidation and its Effects on Uhmwpe
Oxidation and oxidative degradation have been the most pervasive problem in hard-on-soft total joint implant constructions (19,37). Historically, “conventional” UHMWPE implants were gamma sterilized in air directly after consolidation. These implants had high wear, high rates of degradation, and high rates of revision (Fig. 14.2). Gamma sterilization, which comprises a low dose (25 to 40 kGy) of ionizing radiation in the form of gamma radiation, caused some cross-linking but also left behind some long-lived free radicals prone to oxidation in the long term. In 1995, conventional UHMWPE implants started being sterilized while packaged in inert gas (38). The oxidation rate observed in
these implants has been much lower under shelf storage than those sterilized in air; however, the oxidation rate in vivo still is measurable and progressive (39).
these implants has been much lower under shelf storage than those sterilized in air; however, the oxidation rate in vivo still is measurable and progressive (39).
A discussion of our understanding of oxidation and oxidation mechanisms in UHMWPE is crucial in understanding (1) the timescale and effects of oxidation on the wear resistance and mechanical properties of the polymer and (2) the development efforts in improving the oxidation resistance of the polymer.
During ionizing irradiation of UHMWPE, free radicals are formed, the most prevalent of which are the carbon free radicals resulting from the breakage of the C–H bonds (40,41,42,43). Most of these free radicals recombine in the amorphous portion of the polymer (44), where the chains are highly mobile, resulting in cross-linking. This reaction can result in Y-linkages (a midchain free radical with a terminal reactive group) or in H-linkages (two midchain free radicals), which may yield networks with different cross-link structures. The remaining free radicals are trapped in the highly ordered crystalline lamellae (45,46). Because the interchain distance is fixed at 4.1 Å between polyethylene chains in a crystal and the C–C bond distance is 1.5 Å (23), cross-linking in the crystalline phase is highly unlikely. Therefore, free radicals may travel along the polymer chains in the crystals and encounter other free radicals to form a double bond or encounter the crystalline/amorphous interface. The decay of free radicals in the crystalline phase, therefore, is extremely slow, taking as long as years (47).
When oxygen is present in irradiated polyethylene, it reacts with the primary free radicals to form peroxy free radicals (40,48,49,50,51). These peroxy radicals abstract a hydrogen atom from other polyethylene chains, creating primary free radicals, which can then react with oxygen to further this cascade (52,53). When peroxy free radicals get hydrogenated, they form hydroperoxides, which are not stable and, over time, degrade into oxidation products, mainly ketones, esters, and acids (54,55,56,57,58). The formation of these oxidation products is accompanied by chain scission and a decrease in the molecular weight of polyethylene, leading to deterioration of mechanical properties (59,60,61).
Trapped free radicals in irradiated UHMWPE are measured by electron spin resonance (ESR) or electron paramagnetic resonance (EPR) measurements. The interactions of the free radical spins in the sample with the applied magnetic field result in a spectrum comprising signature peaks of different types of free radicals (Fig. 14.3). Typical free radical spectra of conventional UHMWPE shows a 6/7 peak spectrum comprising a combination of alkyl/allyl free radicals. Over time, these free radicals decay into a single peak when in contact with oxygen, the nature of which is debated (51,62).
The reactions of polyethylene free radicals with oxygen and the decay of the formed hydroperoxides eventually result not only in carbonyl-containing species, which are defined as “oxidation” in UHMWPE but also in chain scission, lowering the molecular weight of the material and degrading its material properties (56). Oxidation is determined by spectroscopic techniques measuring carbonyl moieties on UHMWPE formed as a result of the decay of hydroperoxides (63). Typically, accelerated aging methods are used in vitro to compare the oxidative stability of various polyethylene formulations (30). Although accelerated aging is helpful in comparing the oxidation resistance and oxidation potential of different types of bearing materials, it cannot be used to predict the oxidation timeline or profile of a particular material in vivo.
The overall mechanical degradation of UHMWPE is often determined through the determination of its mechanical strength or toughness as a function of oxidation. Although in
vitro studies often use the ultimate tensile strength or fatigue crack propagation resistance as indicators (64), retrievals have limited amount of material and are only amenable to a toughness test named “small punch testing” (65,66). For example, the fatigue strength of UHMWPEs are often measured by measuring their resistance to the propagation of a crack formed under fatigue stress (ASTM E-647, section A1). Using this method, fatigue strength is measured by quantifying a stress factor range at crack inception, ΔKi, whose value ranges from 1.6 to 2.0 MPam1/2 for consolidated UHMWPE without further processing. After accelerated aging of conventional, gamma-sterilized (in air) UHMWPE, oxidation decreased its fatigue strength from 1.29 to 0.18 MPam1/2 (67). Although the rate of degradation in the mechanical properties of UHMWPEs have not been quantified widely in vivo, the overall impact toughness of oxidized conventional retrievals, measured by small punch testing, was shown to decrease (60). It is also known that aside from directly increasing the risk of fracture by decreasing mechanical strength in relevant failure modes, oxidation can also exacerbate fatigue-induced wear mechanisms such as delamination and can deteriorate implant performance without a catastrophic failure (Fig. 14.4).
vitro studies often use the ultimate tensile strength or fatigue crack propagation resistance as indicators (64), retrievals have limited amount of material and are only amenable to a toughness test named “small punch testing” (65,66). For example, the fatigue strength of UHMWPEs are often measured by measuring their resistance to the propagation of a crack formed under fatigue stress (ASTM E-647, section A1). Using this method, fatigue strength is measured by quantifying a stress factor range at crack inception, ΔKi, whose value ranges from 1.6 to 2.0 MPam1/2 for consolidated UHMWPE without further processing. After accelerated aging of conventional, gamma-sterilized (in air) UHMWPE, oxidation decreased its fatigue strength from 1.29 to 0.18 MPam1/2 (67). Although the rate of degradation in the mechanical properties of UHMWPEs have not been quantified widely in vivo, the overall impact toughness of oxidized conventional retrievals, measured by small punch testing, was shown to decrease (60). It is also known that aside from directly increasing the risk of fracture by decreasing mechanical strength in relevant failure modes, oxidation can also exacerbate fatigue-induced wear mechanisms such as delamination and can deteriorate implant performance without a catastrophic failure (Fig. 14.4).
![]() Figure 14.4. Delamination of the articular surface of an oxidized acetabular liner caused by fatigue cracks formed on the subsurface. |
Our understanding of oxidation resistance has been centered on limiting the reactions of the residual free radicals with oxygen over the long term. The decrease in the oxidation rate of gamma-sterilized implants packaged in inert gas during irradiation and shelf storage has been attributed to the decrease in the rates of reaction between polyethylene free radicals and oxygen. Another approach to limiting the reactions of the residual free radicals is eliminating them or decreasing them to undetectable levels before implantation. We will discuss cross-linked UHMWPEs, which are irradiated and melted after irradiation to convert their rigid crystalline regions to flexible amorphous regions for a while to allow the recombination and elimination of these free radicals. When such a method is used, the terminal sterilization method is a nonradiation method such as gas plasma or ethylene oxide sterilization to avoid introducing new free radicals into the polymer before implantation.
Until recently, it was believed that oxidation in vivo was caused largely by radiation-induced free radicals during processing and that the “passive” stabilization of radiation-induced free radicals in UHMWPE, that is, their decrease to undetectable levels at the time of implantation would ensure the oxidative stability of the polymer for the lifetime of the implant. The accumulating data (currently at 7 to 10 years) on irradiated and melted UHMWPEs without free radicals at implantation, where the overwhelming oxidative effect of radiation-induced free radicals is removed, may enable the identification of other possible mechanisms, if any, of oxidation in vivo. Recent findings of small amounts of oxidation in vivo in long-term cross-linked UHMWPEs without detectable free radicals (68) and significant unexpected oxidation in explanted irradiated and melted UHMWPEs stored on the shelf (69) suggested that there may be active oxidation initiating mechanisms in vivo even in the absence of free radicals. In fact, accelerated aging of irradiated and melted UHMWPEs infused with synovial fluid lipids known to absorb into joint implants (70) suggested that the infusion of such lipids into UHMWPE could initiate oxidation in the absence of trapped free radicals (71). An alternative mechanism of oxidation initiation may be cyclic loading and the associated accumulated strain in the polymer in vivo. It was also shown that oxidation in UHMWPEs with detectable free radicals and in irradiated and melted UHMWPEs without free radicals, cyclic loading increased observed oxidation compared to samples aged under the same conditions without cyclic loading (72; Fig. 14.5).
The newest clinically available formulations of UHMWPEs contain antioxidants, the most common of which is vitamin E. Antioxidant-stabilized UHMWPEs were developed from two different paths with the rationales of (1) improving the oxidation resistance of conventional UHMWPE (73,74), and (2) improving the mechanical strength of irradiated and melted cross-linked UHMWPE without sacrificing its wear and oxidation resistance (67). We will describe in detail the development and properties of vitamin E–stabilized UHMWPEs. In view of the recent findings suggesting possible new mechanisms of oxidation in vivo, the inclusion of antioxidants which can protect against free radical reactions in situ may be highly desirable.
Radiation Cross-Linking of Uhmwpe Against Wear
Radiation cross-linking of UHMWPE with high-dose irradiation (>40 kGy) has been the most significant development improving the clinical performance of UHMWPE joint implants (75). Cross-linking methods have been developed to decrease the wear of UHMWPE articular surfaces, which have been the major cause of periprosthetic bone loss or osteolysis in the mid- to long-term lifetime of joint implants since their inception in the 1960s.
Understanding the wear mechanism of UHMWPE became central to the aim of improving the wear resistance of this polymer. The analysis of retrieved acetabular components showed that the highly worn areas contained
numerous multidirectional scratches along with fine, drawn-out fibrils oriented parallel to each other (76). These fibrils were thought to be linked to wear particles observed in periprosthetic osteolytic tissue (77). Thus, wear of UHMWPE appeared to occur through the break-up of fibrils formed by large-strain plastic deformation and orientation of the surface. The fibrils were presumably weakened in the transverse direction to orientation and subsequently ruptured during multidirectional motion of the hip. These in vivo findings were later reproduced in vitro using wear simulators (77,78,79), also revealing that multidirectional motion was part of the underlying mechanism of wear of UHMWPE.
numerous multidirectional scratches along with fine, drawn-out fibrils oriented parallel to each other (76). These fibrils were thought to be linked to wear particles observed in periprosthetic osteolytic tissue (77). Thus, wear of UHMWPE appeared to occur through the break-up of fibrils formed by large-strain plastic deformation and orientation of the surface. The fibrils were presumably weakened in the transverse direction to orientation and subsequently ruptured during multidirectional motion of the hip. These in vivo findings were later reproduced in vitro using wear simulators (77,78,79), also revealing that multidirectional motion was part of the underlying mechanism of wear of UHMWPE.
In early developments, cross-linking of UHMWPE was performed by two different methods: radiation cross-linking and chemical cross-linking (80,81,82). In the early 1970s, Oonishi et al. implanted UHMWPE acetabular cups that were cross-linked using extremely high-dose irradiation (600 to 1,000 kGy) of gamma radiation in the presence of air (83,84,85,86,87,88). The radiographic wear measurements showed average rates of steady-state linear head penetration of 0.006 mm/yr for 600-kGy irradiated UHMWPE, compared to 0.098 mm/yr for ethylene oxide–sterilized conventional UHMWPE (89). Grobbelaar et al. also investigated cross-linking of UHMWPE using up to 800 kGy of gamma radiation in the presence of a sensitizing environment such as acetylene (90). They observed a 30% increase in the abrasion resistance of the cross-linked UHMWPE as was measured by the sand-slurry method.
The use of polyethylenes cross-linked by silane chemistry was first proposed with the hypothesis that cross-linked UHMWPE would exhibit less creep and would contribute to decreased penetration in the early stage of implantation (80,81,91). Silane cross-linked UHMWPE showed a slightly improved creep resistance at high sliding velocities under unidirectional motion compared to uncross-linked UHMWPE. There was no significant difference between the wear rate of uncross-linked and cross-linked UHMWPE but this was because of the lubrication and dynamic conditions they used, which were not in their more clinically relevant form at the time (77,79,92,93,94). Wroblewski et al. (82,95) reported on the wear behavior of 22-mm diameter, silane cross-linked acetabular cups, which were actually injection molded HDPE (19), similar to the material described by Atkinson and Cicek (80,81). Wroblewski’s clinical studies with a minimum of 15-year follow-up on 17 patients showed that after an initial “bedding-in” penetration of 0.2 to 0.41 mm at 2 years, the subsequent average penetration rate (or wear rate) was 0.019 mm/yr (96).
Despite the evidence of improved wear resistance also with peroxide cross-linked UHMWPE, its oxidative stability was of concern (31,97). Although these developments introduced cross-linking as a means of decreasing wear and creep in implant components, the large-scale use of cross-linked UHMWPE in joint implants started in 1999 after development of cross-linking and oxidative stabilization methods using radiation cross-linking.
Radiation cross-linking combined with thermal treatment emerged in late 1990s as technologies to improve the wear and oxidation resistance of UHMWPE acetabular components (97,98,99). The development of these technologies led to a series of new alternate UHMWPE-bearing materials, including irradiated and melted, irradiated and annealed, sequentially irradiated and annealed, and irradiated and mechanically annealed UHMWPEs.
The composite structure of UHMWPE and the interactions between its crystalline and amorphous domains results in a ductile and tough material. However, increased ductility and large-strain deformation are also the underlying
causes of adhesive and abrasive wear of UHMWPE (100). Thus, it is believed that cross-linking reduces wear by decreasing the large-strain plastic deformation of the material under stress (101,102,103,104). On the other hand, the decrease in ductility with increasing cross-linking also decreases the mechanical strength of UHMWPE, often measured by the ultimate tensile strength (ASTM D-638; 105) or the fatigue crack propagation resistance/fatigue strength (ASTM E-647; 105,106). The decrease in ductility can be observed by changes in the fracture surfaces of unirradiated and high-dose irradiated (100 kGy) UHMWPE (Fig. 14.6).
causes of adhesive and abrasive wear of UHMWPE (100). Thus, it is believed that cross-linking reduces wear by decreasing the large-strain plastic deformation of the material under stress (101,102,103,104). On the other hand, the decrease in ductility with increasing cross-linking also decreases the mechanical strength of UHMWPE, often measured by the ultimate tensile strength (ASTM D-638; 105) or the fatigue crack propagation resistance/fatigue strength (ASTM E-647; 105,106). The decrease in ductility can be observed by changes in the fracture surfaces of unirradiated and high-dose irradiated (100 kGy) UHMWPE (Fig. 14.6).
![]() Figure 14.6. The fracture surfaces of (A) unirradiated and (B) 100-kGy irradiated UHMWPE. Note the large amount of buckling and plastic deformation on the unirradiated/uncross-linked UHMWPE. |
The wear of UHMWPE components depends also on the surface roughness to an extent (107). Because of the difference in surface roughness at the UHMWPE and metal counterfaces, the initial wear rate involves the removal of the larger “macroscopic” asperities on the UHMWPE surface, whereas the long-term wear rate is governed by the “microscopic” asperity size of the metal counterface. Thus, surface roughness was observed to increase the wear rate of a variety of UHMWPEs including “conventional” UHMWPEs sterilized by gamma irradiation using low dose (25 to 40 kGy) and cross-linked using higher doses of irradiation (>50 kGy) (108,109,110).
In first-generation cross-linked UHMWPEs widely available for clinical use (111), postirradiation thermal treatment was used to increase the oxidation resistance to reduce or eliminate the residual free radicals trapped in the crystalline domains. One approach was to anneal the irradiated UHMWPE below its peak melting point (112), reducing the free radical concentration by providing more energy to the free radicals and allowing them to recombine to some extent (113). The free radicals were reduced by annealing but this implant was also terminally gamma sterilized, which introduced additional free radicals. In the long term, there was significant oxidation in irradiated and annealed UHMWPE in vivo (114,115,116,117). In spite of this, however, at intermediate follow-up (up to 9 years), the in vivo femoral head penetration rates with irradiated and annealed UHMWPE are less than those for conventional UHMWPE (118,119,120,121) and the clinical effects of this elevated oxidation are not yet manifested (117,122). One study showed fatigue damage at the oxidized rim of explanted irradiated and annealed UHMWPE acetabular liners, although none were revised due to rim damage (115).
The second approach was postirradiation remelting of the crystals to allow for the free radicals to recombine completely. This method reduced the radiation-induced residual free radical concentrations to undetectable levels as measured by ESR and resulted in very low measurable oxidation in accelerated aging tests in vitro (123). At the same time, recrystallization was hindered by the newly formed cross-links, the crystallinity was reduced and therefore, the fatigue strength of radiation cross-linked UHMWPE was further reduced (104
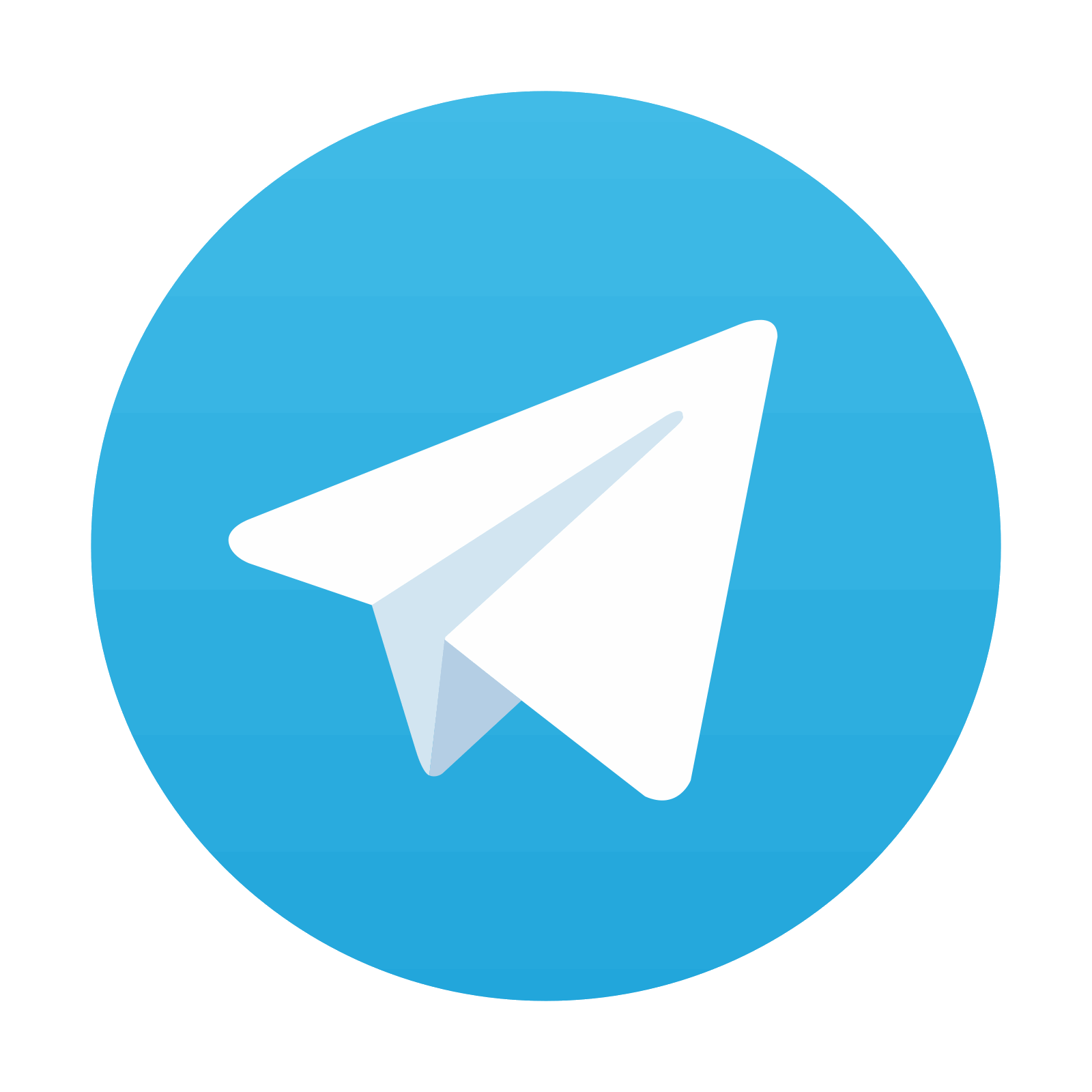
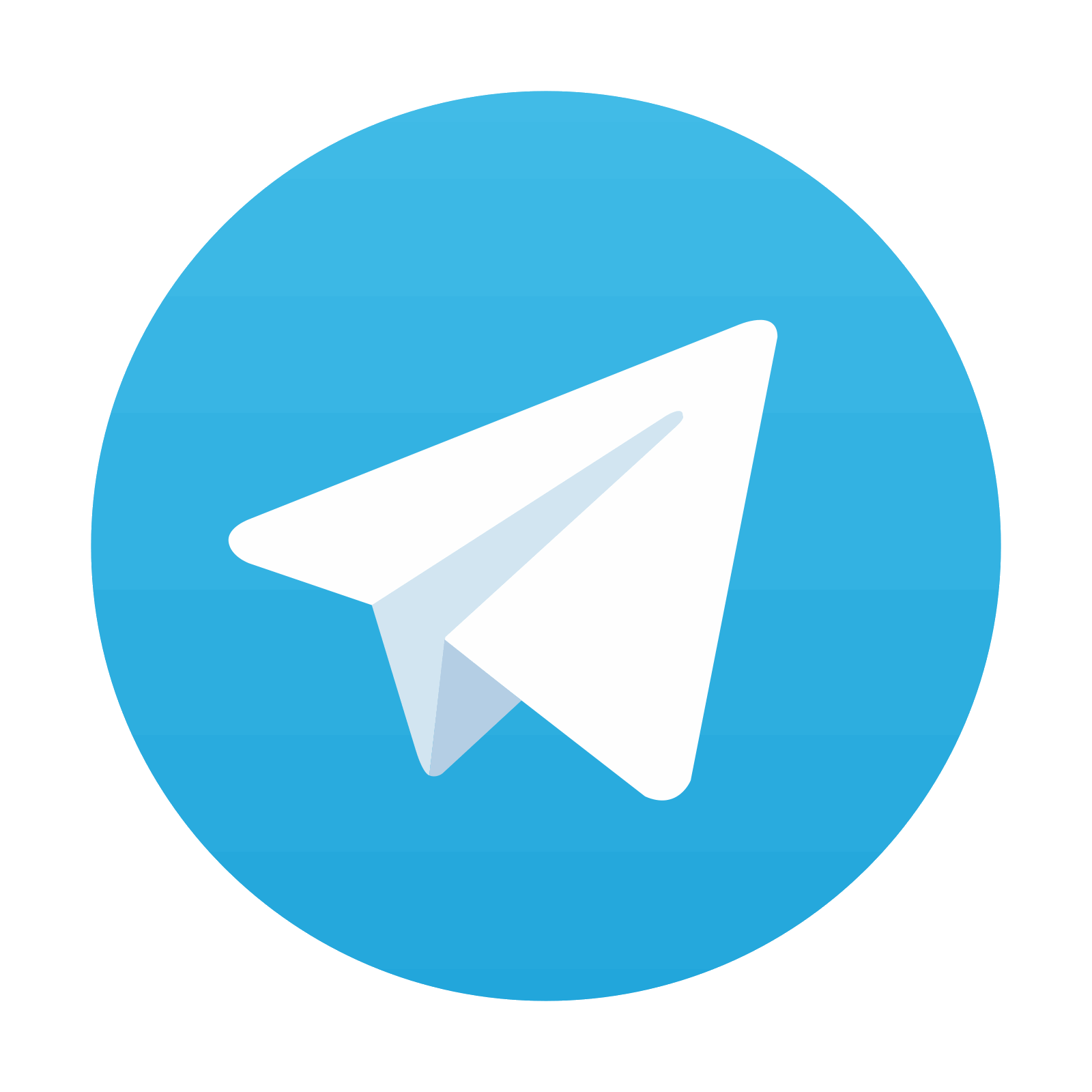
Stay updated, free articles. Join our Telegram channel
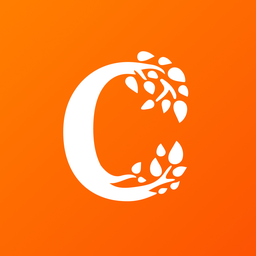
Full access? Get Clinical Tree
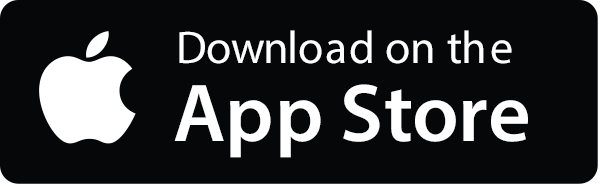
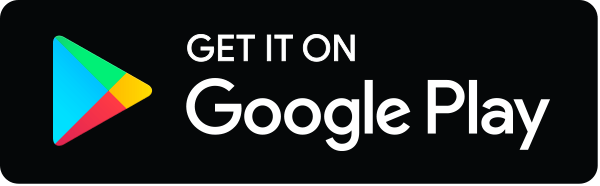