Basic mechanobiology of bone healing
1 Introduction 15
2 Mechanobiology of fracture healing 15
2.1 Intact bone and fracture 15
2.2 Fracture healing 16
2.3 Absolute stability: the rationale of compression osteosynthesis 16
2.4 Relative stability: how much stability is optimal for bone healing? 18
3 Mechanobiology in fracture fixation 21
3.1 General treatment goals 21
3.2 Mechanical and biological aspects of the plate 22
3.3 Mechanical aspects of screws 27
4 Key points 28
5 Summary 29
6 References 29
Introduction
Minimally invasive surgical treatment of skeletal injuries aims to preserve the biology of soft tissue and bone. The rationale for performing mechanical stabilization through fracture fixation is the obvious need to restore anatomy and mechanical function of the bone. Optimal bone healing requires a balance between mechanics and biology and is aided by modern osteosynthesis.
A study of the evolution of fracture management reveals periodic shifting of priorities from biology to mechanics and vice versa. In the early days before the advent of modern fixation techniques the priority of treatment was to achieve solid union of the fracture. Traction and/or external splinting were the preferred methods of treatment even though the mechanical and biological shortcomings of these procedures were obvious. With the development of internal fixation treatment priorities headed towards the opposite extreme, namely the main goal became recovering function by rigidly immobilizing the fracture. The problem was that, all too often, precise reduction and absolute stable fixation were achieved at the expense of extensive soft-tissue trauma caused by the surgery.
To address these issues the development in surgical treatment focused on improvements to the implants and procedures designed to minimize the surgical trauma of open reduction and fixation. Nowadays the damage produced by open surgical approaches has been reduced by the use of minimally invasive osteosynthesis.
The purpose of this chapter is to introduce the basic mechanobiological interactions that occur in bone healing to allow the surgeon to optimize the procedure of fracture stabilization when using minimally invasive osteosynthesis. To achieve improved fracture treatment, the surgeon is encouraged to transfer this knowledge to his/her surgical practice.
Mechanobiology of fracture healing
Intact bone and fracture
The human skeleton provides a protecting and supporting framework for the soft organs. It makes the mechanical functions of the limbs possible and is crucial for locomotion. These functions require the skeleton to be rigid. The bone, as an essential element of the skeleton, provides the necessary rigidity, ie, minimizes load-dependent deformation.
A fracture occurs when the applied force exceeds the strength of the bone. Neighboring elements that were solidly connected separate. Like the phenomenon of cavitation, a rapid dislocation of fracture fragments (within milliseconds) produces a void and results in important trauma to the soft tissue near the fracture site ( Fig 2.1-1 ). The symptoms of loss of function (functio laesa) and pain develop. Mechanically, fracturing leads to local interruption of the bone rigidity and hence abolishes the support function of the bone.

Fracture healing
As an immediate response to a fracture, biological processes are triggered to restore the original function and/or structure of the bone. A fractured bone is considered to be clinically healed when it has recovered stiffness, thus allowing unrestrained function, like weight bearing. In addition complete healing includes remodeling of the scars whereby the woven bone (callus) is replaced by lamellar bone. In this regard, bone is unique because it can regain its original structure without permanent scarring.
Biological mechanisms are complex and cannot be distinctly separated from each other. However, according to a generally accepted opinion [ 1– 3] fracture healing may be divided into arbitrary phases to allow a clearer understanding of the process.
Inflammatory phase (1–7 days postinjury)
Traumatic failure of the bone structure is accompanied by collateral soft-tissue damage. Periosteal and endosteal blood-vessel disruption results in necrotic bone tissue at the fractured bone ends. Chemotactic signals are triggered by platelet degranulation and cytokines are released to initiate angiogenesis.

Repair phase (2 days–6 months postinjury)
As inflammation reduces, a reparative callus forms mechanically stabilizing the injured zone. Under conditions of oxygen deficiency, fibro- and hyaline cartilage will differentiate. Revascularization, considered a fundamental process for bone healing, takes place. Osteogenic factors cause further differentiation of chondroid tissue into bone—a process comparable to the differentiation that occurs at the growth plate. Away from the fracture site intramembranous ossification occurs to create hard fracture callus ( Fig 2.1-2 ). Around the fractured bone ends endochondral ossification occurs to create soft callus. Then calcified cartilage transforms into mineralized bone tissue. The hydroxyapatite density further increases providing enhanced stiffness to the repair construct. This process becomes apparent on x-rays.
Bone remodeling phase (3 months–1 year postinjury)
After achieving “clinical union”, interaction of osteoclasts and osteoblasts leads to replacement of callus and woven bone by mechanically superior lamellar bone. This maturation of the bone tissue includes realignment of osteons. Cancellous bone remodels to form bone trabeculae whereas cortical bone remodels to form a Haversian structure.
Absolute stability: the rationale of compression osteosynthesis
Absolute stabilization of the fracture site results in primary bone healing. Rigid fixation is provided across the fracture site preventing gap motion. Healing occurs with marginal or no callus formation. Danis named this as healing through internal welding (soudure autogène) [ 4]. Biologically the fracture plane is directly bridged. It then remodels into longitudinal Haversian structures to regenerate the bone architecture.
Internal fixation aiming at absolute stability involves applying compression between the fracture planes. Preload suppresses intermittent gapping and prevents shear by friction locking and interdigitation of rough surfaces. Physiologically the compressive preload is superimposed by functional loading. Thus, if load produces additional pressure, the compression of the fracture is amplified. In case of superimposed tension, eg, due to an eccentric functional load vector, the compression is locally diminished ( Fig 2.1-3 ). The fracture surfaces are held closely together as long as the compression is greater than the tension. Should the opposite occur and the tension becomes predominant, the fracture surfaces open up (intermittent instability).

Initially, the concept of absolute stability was criticized because of the argument that bone would undergo pressure necrosis [ 5]. The bone resorption in the vicinity of an aortic aneurysm seemed to prove this point ( Fig 2.1-4a ). However, with an instrumented compression plate it was possible to show that fracture surfaces do not resorb when compression is constantly maintained [ 6]. Further experiments demonstrated that resorption was not a sequela of compression but of intermittent displacement [ 7] ( Fig 2.1-4b–c ). This observation indicated that periodic microseparation of fragments, be it bone-to-bone or metal-to-bone, should be avoided by permanent interfragmental compression to prevent bone loss.

Earlier, implants were thought of as stress protectors. It was felt that a rigid design of the implant would unload the bone leading to so-called “stress protection porosis.” This temporary loss of bone was erroneously attributed to mechanical unloading according to Wolff‘s law. However, if it was felt that Wolff‘s law explained the short-term loss of bone due to unloading, it would be difficult to understand why, with ongoing absence of loading, the porosis disappeared after a few months ( Fig 2.1-5 ). The phenomenon is in fact a temporary porosis related to the process of internal remodeling and removing dead bone.
Relative stability: how much stability is optimal for bone healing?
While the degree of stability is clearly defined when compression fixation is used, the degree of flexibility needed with flexible fixation is harder to define. The concept of relative stability involves controlled gap motion because the implant used is flexible ( Fig 2.1-6 ). Initially, internal fixation devices were mainly perceived as compressing stress-protectors to prevent fracture mobility (absolute stability). The appearance of fracture callus was considered a symptom of lack of stabilization. In contrast, the concept of relative stability takes advantage of callus formation. This process is called indirect healing. Callus prepares a healing environment by stabilizing the fracture, before, in a second phase, solid bridging takes place. Extensive research in the past decades revealed that mechanical stimulus accelerates healing processes which makes relative stability an important pillar of modern osteosynthesis [ 8– 10].


The macroscopic loading environment at a fracture site may be complex. The predominant compression force is often superimposed with bending and shear (torsion) leading to a specific reversible deformation of the fracture gap. However, understanding the influence of load on bone healing involves a closer look at the cellular level. It is crucial to understand that the important parameter is not the macroscopic motion within the fracture, but the deformation of the repair tissue at the level of the single cell. The relevant stimulus on a cell may be its individual deformation either compressed or tensioned. The mechanical macroenvironment has an immediate impact on the mechanical environment of a cell (microenvironment) and is influenced by two major factors [ 11]:
Gap motion: the displacement of the fracture surfaces in relation to each other.
Gap width: the initial distance between the fracture surfaces.
The technical term “strain” describes deformation of a body in relation to its dimension. “Strain” seems the best way to measure tissue deformations by relating the macroscopic gap motion to the gap width (gap motion divided by gap width). A given displacement within a fracture gap is equally “shared” by the cells bridging that gap. With a decreasing number of cells (decreasing gap width), each cell experiences a larger individual deformation (increasing strain) ( Fig 2.1-7 ). For example, in a hairline fracture of 20 µm (one cell layer wide) the repair tissue is already deformed 100% at a gap displacement of only 20 µm. This condition could disable or delay healing (see 2.3 Absolute stability: the rationale of compression osteosynthesis). According to Yamada, liver parenchyma, here assumed to offer similar properties to granulation tissue, fails at 100% elongation [ 12]. Cartilage ruptures at 10% and cortical or cancellous bone at 2% elongation ( Fig 2.1-8 ).
In addition to the size of the fracture gap geometric structuring profoundly affects the mechanical behavior of repair tissues. For example, a spring made of metal or tissue is able to provide larger overall deformation at a specific deflection of its finite element compared with a directly bridging body. It is noteworthy that the bone callus, called “woven bone” due to its three-dimensional structure, tolerates higher strain (up to 10%) than one might expect if an unstructured material were to connect the fracture surfaces directly.


It can be safely assumed that tissue cannot be formed under conditions where it would be disrupted. For example, bone cannot be generated when the local tissue strain exceeds 2%. The goal of flexible fixation is to create a situation where the deformation of the repair tissue that is present between the fracture ends remains tolerable when the fracture is loaded. In cases where the gap mobility cannot be controlled, the gap width must be adjusted to obtain acceptable conditions for bone healing. Initial bone resorption (see 2.3 Absolute stability: the rationale of compression osteosynthesis) at the fracture ends may be regarded as a natural response of the biological system to reduce tissue strain by enlarging the fracture width. Solid healing can occur even in medically untreated cases under conditions of large fracture displacement when the distance between the fracture fragments is also large. On the other hand, below a certain limit of strain no bone healing is induced, which, among other factors, might help to explain why a large, splinted gap does not heal.
The experiments of Hente et al provide an interesting insight into the effect of strain on fracture healing [ 13]. In the experimental model a strain gradient was applied from 0% to 90% along a fracture plane by changing the gap displacement ( Fig 2.1-9 ). Histological sections at distinct strain levels revealed solid bone bridging of the gap and moderate callus formation at 5% strain. At 30% strain more callus was induced but no bridging became obvious, while 65% strain revealed massive callus formation without bridging. This experiment has implications for plate osteosynthesis. Due to the eccentric position of the plate with respect to an idealized functional load vector, the fracture gap deformation describes a gradient from small deflection close to the plate to large deflection far from the plate. The tissue deformation can therefore not be regarded as constant when plating a fracture but varies with its location ( Fig 2.1-10 ). These theoretical considerations are supported by observing the callus formation of a plated fracture ( Fig 2.1-6a ). Besides callus in the medullary cavity, periosteal callus is generated at the far cortex from the plate. Periosteal callus mainly forms opposite the plate always supporting the unstable region.
As mentioned, the second parameter influencing tissue strain is the motion that occurs in the fracture gap. A functional load is the initiator of gap mobility. This is dependent on patient activity. To prevent overload at the fracture site, the degree of activity of the patient needs careful monitoring during rehabilitation.


With functional load the resulting mobility in the fracture is controlled by the stiffness of the repair construct consisting of bone and fixation devices. Herein lies the relevance of mechanobiology to osteosynthesis. Bone healing can be actively influenced by controlling both the mechanical properties and the configuration of the fixation elements (see 3.2 Mechanical and biological aspects of the plate). Callus will form to counteract tissue deformation and thereby unload the implant. Flexible fixation creates a load-sharing situation between newly formed bone tissue and implants. When achieved the biology regulates the tissue deformation over the healing period.
The application of this knowledge to clinical fracture treatment using flexible splinting must take into consideration gap motion and gap width which both influence the amount of strain that occurs at a fracture gap. The strain tolerance of repair tissue is the critical factor and the gap width and gap motion must be controlled so that this tolerance is not exceeded.
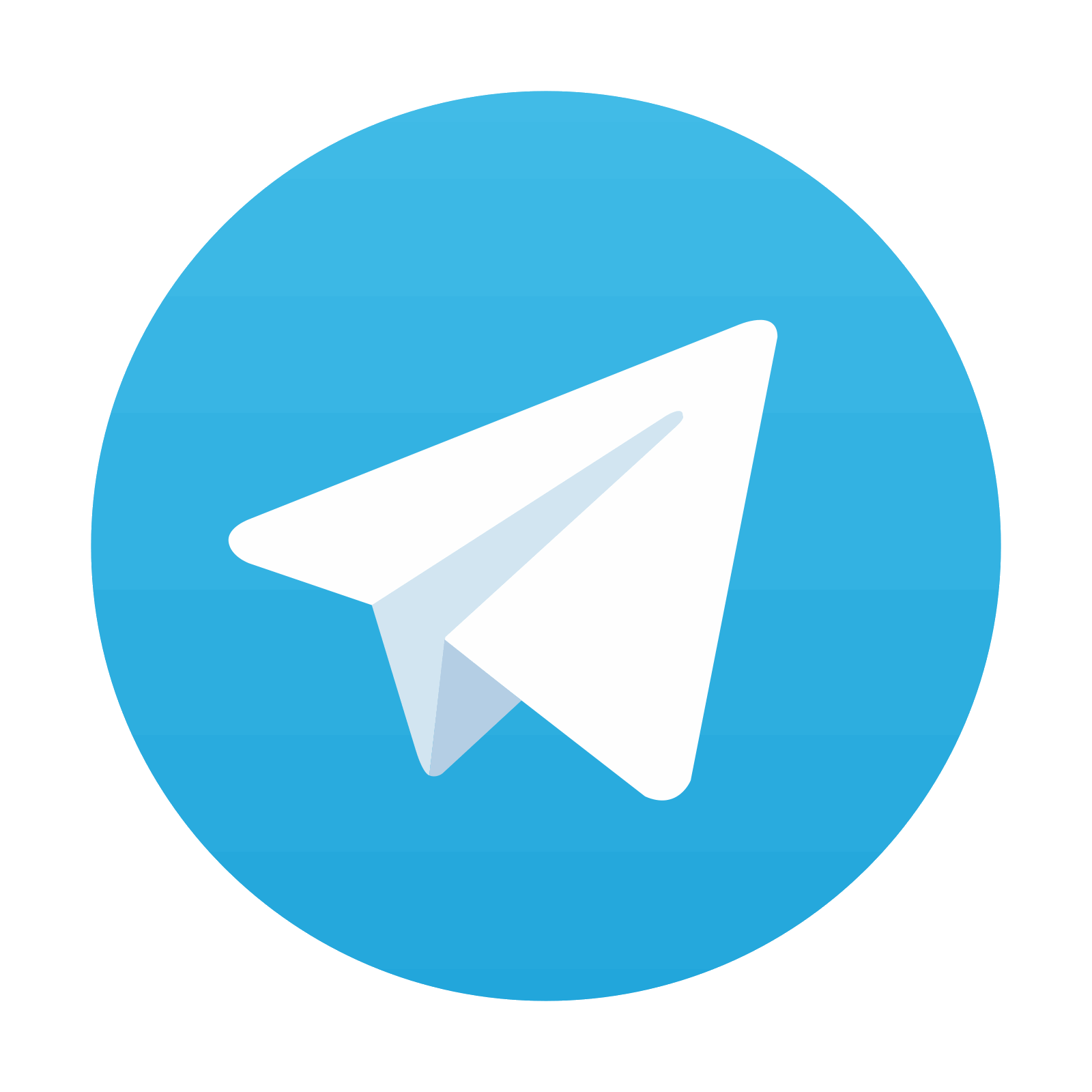
Stay updated, free articles. Join our Telegram channel
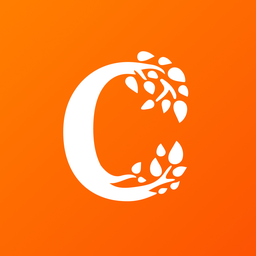
Full access? Get Clinical Tree
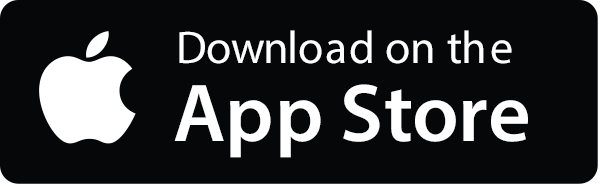
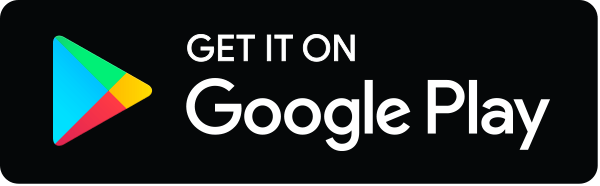
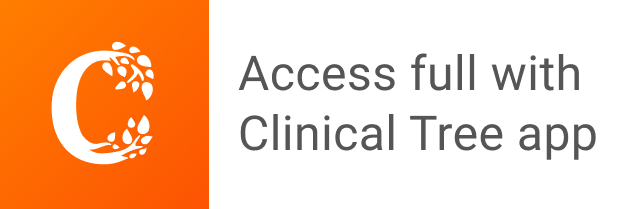