The autonomic nervous system (ANS) plays a key role in the regulation of many physiologic processes, mediated by supraspinal control from centers in the central nervous system. The role of autonomic dysfunction in persons with spinal cord injuries is crucial to understand because many aspects of the altered physiology seen in these individuals are directly caused by ANS dysregulation.
The intact spinal cord is responsible for carrying and modulating many types of signals, both those of the somatic nervous system, which is involved in motor and sensory processes, but also the myriad of functions occurring under the control of the autonomic nervous system (ANS). After spinal cord injury (SCI), damage to the autonomic pathways that travel in the spinal cord leads to altered regulation of many processes that are subsumed by the autonomic nervous system. The ANS regulates many functions, including control of cardiovascular functions such as coronary blood flow, cardiac contractility, heart rate, and peripheral vasomotor responses. In addition, the autonomic nervous system controls blood flow to skeletal muscle, kidneys, the splanchnic circulation, and the skin. Impaired ANS regulation caused by SCI leads to many of the clinical issues seen in persons with SCI, including altered cardiovascular and thermoregulatory function and manifestations of end-organ dysfunction, such as neurogenic bowel and bladder. This review focuses mainly on the effects of autonomic nervous system dysfunction on cardiovascular control in individuals with SCI.
Elements of autonomic nervous system dysfunction after SCI can be divided into acute and chronic processes, with some overlap. Acutely, neurogenic shock occurs with its component hypotension, hypothermia, and bradycardia. Hypotension and bradycardia last 2 to 6 weeks and are accompanied by arrhythmias that occur primarily in the acute setting. Pulmonary effects of disruption of sympathetic innervation in persons with tetraplegia also are of importance in the period immediately after injury. Orthostatic hypotension occurs acutely but is clinically more relevant during the postacute phase during rehabilitation when mobilization is occurring. However, some patients have long-term orthostasis. Chronic manifestations of autonomic dysfunction include impaired temperature regulation and impaired cardiovascular function and responses to exercise. Autonomic dysreflexia is also caused by altered autonomic nervous system function but will not be covered in this review.
Autonomic nervous system anatomy
The ANS has two components or divisions that may be disrupted as a result of damage to the spinal cord, the parasympathetic nervous system (PNS) and the sympathetic nervous system (SNS). The enteric nervous system, also considered a division of the ANS, is not directly disrupted after SCI. The level and extent of injury to the spinal cord determines the dysfunction that occurs. A thorough understanding of the anatomy is important in predicting the types of alterations in function that will occur after spinal cord injury.
The autonomic nervous system controls many functions via complex pathways, typically with the sympathetic division and parasympathetic division serving opposing regulatory roles. These reflex pathways consist of sensory receptors, afferent pathways, integration centers in the central nervous system (CNS), efferent pathways, and effector organs . Ascending and descending impulses are generally carried in the spinal cord (with the exception of the cranial parasympathetic division) and connect with interneurons, which begin in the CNS, traverse the ventral roots, and terminate in an ANS ganglion outside the CNS. These interneurons are called preganglionic neurons , and the effector neurons that originate in the ANS ganglion are called postganglionic neurons . Both divisions of the autonomic nervous system have preganglionic and postganglionic neurons. The cell bodies of the preganglionic neurons lie in the brain or spinal cord. The cell bodies of the postganglionic neuron lie in autonomic ganglia, which are located either near the spinal cord or near the effector organ. The viscera are innervated by both divisions of the ANS, which typically provide opposing regulatory controls. However, some blood vessels and sweat glands have only a single type of innervation.
The PNS has cell bodies in the brainstem and sacral spinal cord and thus is often referred to as the craniosacral division of the ANS . The preganglionic fibers arise in the visceral brainstem nuclei and the second through fourth sacral segments. The cranial nerve fibers are carried with cranial nerves III, VII, IX, and X and innervate structures in the head and neck as well as thoracic and abdominal viscera. The preganglionic parasympathetic fibers that innervate the descending colon and pelvic organs originate in the sacral segments. The spinal cord has the important role of carrying efferent impulses from the brainstem via the lateral reticulospinal tract to the sacral cord. Most preganglionic parasympathetic fibers synapse in the inferior mesenteric ganglion, but some continue without synapsing through the hypogastric nerves to reach the vesical plexus in the bladder wall .
The SNS has cell bodies in the intermediolateral or intermediomedial cell columns of the spinal cord, and the preganglionic fibers leave the cord and join the ventral roots of T1 to L2. Hence, this division is often referred to as the thoracolumbar division . Variations in level of origin do occur, and preganglionic fibers from as high as C7 to as low as L4 have been shown . Preganglionic fibers typically run in the spinal cord for several levels, and in fact some remain intraspinal for up to 12 levels. In addition, some of the fibers cross while others remain uncrossed. In general, axons destined for thoracic ganglia tend to be unilateral, whereas those going to lumber ganglia are typically bilateral.
Preganglionic sympathetic fibers may synapse in the paravertebral ganglia of the sympathetic chain located on either side of the vertebral bodies or in collateral (prevertebral) ganglia located near the viscera . Above the diaphragm, all sympathetic preganglionic fibers synapse in the sympathetic chain. In the cervical region, there are several paravertebral ganglia, namely the superior cervical ganglion, the middle cervical ganglion, and the stellate ganglion (consists of the inferior cervical ganglion and first thoracic ganglion) . In the thoracic region there are up to 11 paravertebral ganglia. There are four lumbar and four sacral paravertebral ganglia. The chain ganglia in the coccygeal region are fused into the coccygeal ganglion or ganglion impar.
Preganglionic sympathetic fibers to the abdominal and pelvic viscera travel through the paravertebral ganglia without synapsing and then form splanchnic nerves. These fibers synapse with the postganglionic neurons, which are located in collateral ganglia, and the fibers then travel with major arteries to the effector organs. The superior and infection mesenteric ganglia are examples of collateral ganglia in which pre- and postganglionic fibers synapse. The postganglionic fibers of the SNS also form visceral nerves, such as the cardiac nerves, and join peripheral nerves to innervate blood vessels and the skin.
The heart is innervated by both sympathetic and parasympathetic postganglionic fibers. Cardiac sympathetic preganglionic neurons synapse with postganglionic neurons in the middle cervical ganglia and stellate ganglion . Cardiac parasympathetic fibers originate in the dorsal motor nucleus of the vagus and the nucleus ambiguous in the medulla oblongata and travel in the recurrent laryngeal nerves and vagus nerve . These nerves interconnect with sympathetic cardiopulmonary nerves near the pulmonary artery to form the ventral and dorsal cardiopulmonary plexuses . Emerging from these plexuses are three large cardiac nerves as well as several small cardiac nerves, which serve to innervate the conduction system, the atria, and the ventricles. Parasympathetic fibers synapse with postganglionic cells on the epicardial surface or within the walls of the heart near the sinoatrial (SA) or atrioventricular (AV) node.
Autonomic nervous system physiology
The autonomic nervous system regulates all essential physiologic components of circulation including heart rate, stroke volume, and vascular resistance, which, in turn, determine arterial blood pressure and cardiac output. The autonomic nervous system regulates these functions in response to feedback from afferent pathways by affecting the force and frequency of cardiac contraction and vasodilation or vasoconstriction. Other influences have a role in the final physiologic state, including hormonal and local metabolic factors and intravascular volume.
Control of cardiovascular function is via a complex system of feedback loops that modulate sympathetic and parasympathetic input. Arterial chemoreceptors, ergoreceptors in skeletal muscle, and cardiopulmonary receptors with sympathetic afferents provide excitatory input to the nucleus tractus solitarius . Parasympathetic afferents transmit information from the aortic arch and carotid baroreceptors as well as receptors in the systemic and pulmonary vessels, the great veins, and the atria. These afferent impulses travel in cranial nerve IX (glossopharyngeal) and X (vagus) to provide inhibitory input to the nucleus tractus solitarius .
The arterial baroreceptors located in the aortic arch and carotid sinuses respond to a reduction in arterial pressure by decreasing activity of the parasympathetic nerves and increasing activity of the sympathetic excitatory nerves. In contrast, the baroreceptors respond to an increase in arterial pressure by stimulation of the spinal sympathetic inhibitory tract, inhibition of the spinal sympathetic excitatory tract, and stimulation of the activity of parasympathetic preganglionic nerves . Via these feedback controls, the sympathetic outflow is modulated, with parasympathetic outflow serving an inhibitory role.
Heart rate and rhythmicity are also under the control of the autonomic nervous system. The SA node is under direct influence of both the SNS and PNS. Sympathetic input increases the rate at which the SA node generates action potentials, and parasympathetic input decreases the rate of action potential generation .
The SNS exerts primary control of blood flow to the skin, kidneys, and splanchnic organs, whereas local metabolic influences exert primary control over blood flow to the heart and skeletal muscle . The splanchnic circulation supplies blood flow to the abdominal organs, including the gastrointestinal tract, spleen, pancreas, and liver. These organs receive about 25% of resting cardiac output and contain more than 20% of circulating blood volume. The arteries and veins of these organs are richly innervated with sympathetic vasoconstrictor nerves, thus, activation of the SNS can produce an 80% reduction in flow in the splanchnic circulation and cause a large shift of blood to the central circulation . Renal blood flow also decreases during SNS activation, and low perfusion pressures are thought to trigger the release of renin.
The final outcome of all these processes is regulation of systemic vascular resistance and cardiac filling pressure (and thus stroke volume and cardiac output), which, in turn, control arterial blood pressure. Table 1 delineates the sympathetic outflow by effector organ, receptor, neurotransmitter type, and function (data from Ref. ).
Target organ or structure | Heart: coronary arteries, sinus node, conduction system | Kidney: juxtaglomerular cells | Systemic resistance vessels | Capacitance vessels: primarily splanchnic | Adrenal medulla |
---|---|---|---|---|---|
Adrenoreceptor type | Beta | Beta | Beta | Beta | Alpha |
Neurotransmitter | NA | NA | NA | NA | ACh |
Function | Regulates heart rate, cardiac contractility, coronary blood flow | Release of renin | Vasoconstriction: Regulates SVR | Vasoconstriction: Regulates cardiac filling pressure and stroke volume | Release of circulating adrenaline, which also activates vascular β-receptors. |
Autonomic nervous system physiology
The autonomic nervous system regulates all essential physiologic components of circulation including heart rate, stroke volume, and vascular resistance, which, in turn, determine arterial blood pressure and cardiac output. The autonomic nervous system regulates these functions in response to feedback from afferent pathways by affecting the force and frequency of cardiac contraction and vasodilation or vasoconstriction. Other influences have a role in the final physiologic state, including hormonal and local metabolic factors and intravascular volume.
Control of cardiovascular function is via a complex system of feedback loops that modulate sympathetic and parasympathetic input. Arterial chemoreceptors, ergoreceptors in skeletal muscle, and cardiopulmonary receptors with sympathetic afferents provide excitatory input to the nucleus tractus solitarius . Parasympathetic afferents transmit information from the aortic arch and carotid baroreceptors as well as receptors in the systemic and pulmonary vessels, the great veins, and the atria. These afferent impulses travel in cranial nerve IX (glossopharyngeal) and X (vagus) to provide inhibitory input to the nucleus tractus solitarius .
The arterial baroreceptors located in the aortic arch and carotid sinuses respond to a reduction in arterial pressure by decreasing activity of the parasympathetic nerves and increasing activity of the sympathetic excitatory nerves. In contrast, the baroreceptors respond to an increase in arterial pressure by stimulation of the spinal sympathetic inhibitory tract, inhibition of the spinal sympathetic excitatory tract, and stimulation of the activity of parasympathetic preganglionic nerves . Via these feedback controls, the sympathetic outflow is modulated, with parasympathetic outflow serving an inhibitory role.
Heart rate and rhythmicity are also under the control of the autonomic nervous system. The SA node is under direct influence of both the SNS and PNS. Sympathetic input increases the rate at which the SA node generates action potentials, and parasympathetic input decreases the rate of action potential generation .
The SNS exerts primary control of blood flow to the skin, kidneys, and splanchnic organs, whereas local metabolic influences exert primary control over blood flow to the heart and skeletal muscle . The splanchnic circulation supplies blood flow to the abdominal organs, including the gastrointestinal tract, spleen, pancreas, and liver. These organs receive about 25% of resting cardiac output and contain more than 20% of circulating blood volume. The arteries and veins of these organs are richly innervated with sympathetic vasoconstrictor nerves, thus, activation of the SNS can produce an 80% reduction in flow in the splanchnic circulation and cause a large shift of blood to the central circulation . Renal blood flow also decreases during SNS activation, and low perfusion pressures are thought to trigger the release of renin.
The final outcome of all these processes is regulation of systemic vascular resistance and cardiac filling pressure (and thus stroke volume and cardiac output), which, in turn, control arterial blood pressure. Table 1 delineates the sympathetic outflow by effector organ, receptor, neurotransmitter type, and function (data from Ref. ).
Target organ or structure | Heart: coronary arteries, sinus node, conduction system | Kidney: juxtaglomerular cells | Systemic resistance vessels | Capacitance vessels: primarily splanchnic | Adrenal medulla |
---|---|---|---|---|---|
Adrenoreceptor type | Beta | Beta | Beta | Beta | Alpha |
Neurotransmitter | NA | NA | NA | NA | ACh |
Function | Regulates heart rate, cardiac contractility, coronary blood flow | Release of renin | Vasoconstriction: Regulates SVR | Vasoconstriction: Regulates cardiac filling pressure and stroke volume | Release of circulating adrenaline, which also activates vascular β-receptors. |
Autonomic nervous system control of thermoregulation
Thermoregulatory responses depend on cold and warm sensors in the hypothalamus and the skin but also on input from mesencephalic, medullary, spinal, and intra-abdominal temperature sensors . Afferent fibers from the peripheral receptors with cell bodies in the dorsal root ganglia enter the spinal cord and ascend contralaterally to the medial lemniscus and the thalamus and then project further to the hypothalamus . The preoptic area of the hypothalamus controls the thermal set-point and integrates thermoregulatory responses . The efferents from the hypothalamus control thermoregulatory vasomotor and sudomotor tone as well as nonshivering and shivering thermogenesis via descending noradrenergic and cholinergic fibers that exit the spinal cord below C-7 .
Sympathetic nervous system control of the skin contributes to thermoregulation. At rest, the skin receives 6% of resting cardiac output. This can be reduced markedly if heat needs to be retained and increase up to seven times normal if heat needs to be dissipated. A reduction in sympathetic vasoconstrictor tone causes vasodilation, because the venous plexus in the skin is richly innervated with sympathetic vasoconstrictor nerves .
When the body is exposed to temperatures greater than 30°C, there is progressive cutaneous vasodilation, which enhances heat loss to the environment . Heat loss by evaporation provides the major physiologic defense against overheating. Sweat glands, which are controlled by cholinergic sympathetic nerve fibers, secrete large quantities of hypotonic saline in response to heat stress. As the sweat reaches the skin, a cooling effect occurs as the fluid evaporates, and the blood that has been shunted from the interior to the surface of the skin is then cooled. The effectiveness of evaporative heat loss is determined by relative humidity. In high humidity, sweating represents a useless water loss and can lead to a dangerous state of dehydration and overheating .
In contradistinction, the initial result of cold exposure is a generalized reduction in skin temperature, which leads to cutaneous veno- and vasoconstriction . A cold-induced increase in peripheral arterial resistance is initiated by beta-adrenergic receptor activation, which acts directly on blood vessel diameter. In addition, sudden cold exposure causes the release of norepinephrine, epinephrine, and cortisol from the adrenal gland . The resultant reduction in peripheral blood flow redirects blood to the central circulation, which results in a rise in mean arterial pressure, cardiac output, and stroke volume.
Heat production via shivering thermogenesis is initiated once there is a significant reduction in body temperature. The contractile force generated by the shivering muscles may be 15% to 20% of that elicited during maximal voluntary muscle activation . It is mediated by somatic nerves and requires an intact spinal cord and posterior hypothalamus . Nonshivering thermogenesis, an increased metabolic heat production from sources other than muscular contraction, also occurs during cold stress. The sources in animals include both an elevated rate of aerobic metabolism of brown adipose tissue as well as the stimulating effects of metabolic hormones .
Alterations in autonomic nervous system function after spinal cord injury
Spinal cord injury results in a reduction or lack of autonomic control that is directly related to the level of injury. Spinal cord injury interrupts the connections between the supraspinal regulatory centers and the effector organs and interferes with both afferent and efferent signal transmission. Many of the major cardiovascular functions are associated with segmental outflow from several levels, such as the outflow to the heart or the splanchnic outflow. Sympathetic control of blood vessels in the limbs and skin, as well as sweat glands, tends to be regionalized to more limited levels of the spinal cord (see Table 2 for synopsis).
CNS or Spinal cord level | ANS division | Ganglion | Nerve | Organs innervated |
---|---|---|---|---|
Dorsal motor nucleus of cranial nerve X | PNS | Not applicable |
| Heart, lungs, abdominal viscera, ascending and transverse colon |
T1–T4 | SNS | Middle cervical and stellate | Cardiac nerves | Heart, Lungs |
T3–L3 (but mainly T5-T9) | SNS | Superior mesenteric (but does not synapse) | Lesser splanchnic nerve | Adrenal medulla |
T5–T11 | SNS | Celiac and superior mesenteric | Greater and lesser splanchnic nerves |
|
L1–L3 | SNS | Inferior mesenteric | Lumbar splanchnic nerves | Descending colon and rectum, kidney, bladder, uterus, external genitalia |
S2–4 | PNS | Not applicable | Pelvic splanchnic nerves | Descending colon and rectum, bladder, uterus, external genitalia |
Supraspinal control of the SNS originates in the rostroventrolateral (RVL) medulla, which is the main sympathetic regulatory center in the CNS . Descending sympathetic input from this supraspinal center travels through the cervical spinal cord and synapse via spinal interneurons with the sympathetic preganglionic neurons starting at T1. Thus, spinal cord injury above T1 interrupts the conduction of signals from the medulla to the thoracic spinal cord. These changes are more significant with more severe injuries . Autopsies findings in patients with cervical SCI and severe cardiovascular abnormalities showed marked loss of axons in the dorsal aspects of the lateral funiculus, which is thought to be the location of the descending vasomotor pathways .
The sympathetic innervation to the heart, including the myocardium, SA, and AV nodes, is from T1 to T4. Thus, injuries between T1 and T4 have partial innervation to the heart, and injuries below T4 gain complete innervation to the heart. Thus, if the level of injury is below T4, normal cardiac responses are maintained, but vascular tone and control of blood pressure will still be under local regulation. Because the parasympathetic innervation to the heart is via the vagus nerve, which does not travel in the spinal cord, the only supraspinal control of cardiac responses is parasympathetic in nature.
Sympathetic outflow to the splanchnic organs mainly originates from T5 to T9 via the greater splanchnic nerve to the celiac ganglion (although there are splanchnic efferents from T5 to L2). This outflow regulates most of the blood flow in the splanchnic circulation. Injuries below T5 have some ability to regulate splanchnic flow, with more control with distal levels of injury. Damage to the spinal cord above this outflow hinders the ability of the splanchnic beds to vasodilate, which allows blood to pool in the splanchnic circulation. This lack of compensatory response to an elevation in blood pressure is part of the pathogenesis of autonomic dysreflexia, which occurs in persons with SCI above T6.
The sympathetic efferents to the adrenal medulla are from T3 to L3, but the major outflow is T5 to T9. This innervation allows control of the release of epinephrine from the adrenal medulla, which is part of the normal response to exercise or stress. Thus, injuries above T9 will have an impaired adrenal response to exercise. Sympathetic efferents to the blood vessels of the lower extremities travel through the upper lumber sympathetic ganglia; thus, vasomotor responses of the lower extremity vessels are abnormal with levels of injury above T12. Only injuries below L1 have minimal effects of SNS dysregulation.
Sympathetic control of blood vessels in the limbs, skin, and sweat glands are more regionalized than the major outflows and functions listed above. Sweat glands receive dual innervation from both cholinergic and adrenergic fibers; however, cholinergic stimulation provokes the largest response . Spinal segments T2 to T4 supply sweat glands on the head and neck, T2 to T8 to glands of the upper limbs, T6 to T10 to the trunk, and T11 to L2 to the lower extremities . Autonomic dermatomes overlap several segments above and below the somatic levels.
After injuries above T1, with loss of supraspinal connections to the sympathetic nervous system, homeostasis is achieved via local and spinal reflex control. There is evidence that after SCI, spinal circuits are capable of generating some sympathetic activity . Sympathetic preganglionic neurons show spontaneous activity after SCI in the absence of input and function as “spinal sympathetic interneurons” . There is also peripheral alpha-adrenoceptor hyperresponsiveness, which is thought to contribute to the propensity for autonomic dysreflexia. Sympathovagal balance appears to be maintained, secondary to a decrease in parasympathetic activity that parallels the decline in sympathetic activity .
Sympathetic preganglionic neurons show morphologic changes after spinal cord injury because of denervation from a lack of descending input. This leads to low resting blood pressure, orthostatic hypotension, and loss of diurnal fluctuation of blood pressure . This decrease in the diurnal variation of blood pressure in persons with cervical SCI is because normal nocturnal decreases in blood pressure do not occur . In addition, the 24-hour plasma noradrenaline level in individuals with SCI also shows little diurnal variation. Interestingly, both sympathetic and parasympathetic preganglionic neurons in the lumbar and sacral spinal cord are less severely affected than those in the thoracic cord and seem to be more dependent on innervation from spinal interneurons for synaptic input .
Cardiovascular alterations immediately after spinal cord injury
In the first few minutes after SCI, there is a disruption in central sympathetic control caused by interruption of the descending pathways that travel in the spinal cord. Animal studies have found that within seconds to minutes after injury, there is a systemic pressor response that results from a burst of sympathetic activity and outflow of adrenaline from the adrenal glands . In the feline model of SCI, compression of the cervical spinal cord causes a brief increase in both systolic and diastolic blood pressure, with corresponding bradycardia . In addition, electrocardiogram changes include ventricular ectopy and a left ventricular strain pattern. Tibbs and colleagues used a canine model in a series of studies showing that spinal cord transection creates an initial increase of norepinephrine, with associated hypertension, increased systemic vascular resistance, increased left ventricular ejection fraction, and bradycardia with escape arrhythmias . Guha and Tator used rats with a clip compression injury model, and showed that T1 SCI is characterized by a brief hypertensive peak (2 to 3 minutes in their model). All investigators noted that profound hypotension followed this initial hypertensive episode.
After this initial brief pressor response, studies of acute SCI in both humans and animals show an extended period of neurogenic shock characterized by hypotension, bradycardia, and hypothermia . Neurogenic shock is a direct result of the loss of supraspinal control and reduction in sympathetic activity below the level of injury, which is substantiated by low plasma adrenaline, noradrenaline, and urinary metabolites after SCI . Neurogenic shock is part of the spinal shock syndrome, which is the period after injury characterized by a marked reduction or abolition of sensory, motor, or reflex function of the spinal cord below the level of injury .
Studies in the feline model of SCI show markedly decreased cardiac sympathetic tone. The loss of direct sympathetic influence on the heart appears to be the primary reason for decreased cardiac output, with a marked decrease in both heart rate and contractility . Hypotension and bradycardia also occurs in the rat model of SCI . This is thought to be because of the strong cholinergic input from unopposed vagal tone leading to nitric oxide release and vasodilation . In addition, vagal stimulation may also depress cardiac function by slowing atrioventricular conduction and altering the synchronicity of the atrial and ventricular contractions, which impairs ventricular filling. Also cholinergic influences depress atrial contractility by antagonizing adrenergic influences on the heart.
The extent and severity of the blood pressure and heart rate changes appears to correlate with the location and severity of the spinal cord injury. Lehmann and colleagues studied 71 persons after SCI, and found that 68% of individuals with complete tetraplegia (21 of 31) had hypotension (defined as systolic blood pressure <90 mm Hg), whereas none of the motor incomplete tetraplegics nor paraplegics met the criteria for hypotension. Delineating the effects of acute sympathetic nervous system withdrawal from hemodynamic shock can be difficult, especially in patients with multitrauma. Thus management must take into consideration both scenarios.
The pathophysiology of acute SCI is thought to be in part caused by spinal cord ischemia. This ischemia may be caused by local factors such as the direct effects of the SCI and focal vasospasm, both of which lead to loss of autoregulation of spinal cord blood flow. In addition, systemic factors such as hypotension also lead to decreased spinal cord blood flow and perfusion. This is compounded by the effects of bradycardia, arrhythmias, reduced mean arterial pressure (MAP), reduced pulmonary vascular resistance (PVR), and decreased cardiac output. Several studies have looked at maintenance of adequate systolic blood pressure as an important way to improve prognosis after acute SCI, based on the reduction of cord ischemia .
Hypotension
In recently injured persons with cervical spinal cord injury, the supine MAP averages 57 mm Hg compared with 82 mm Hg in supine non–cord-injured persons .
In the recently published guidelines on blood pressure management after acute SCI, the conclusion based on review of existing Class III evidence is that providing blood pressure support to keep the MAP >85 to 90 mm Hg improves neurologic outcomes . The duration of blood pressure support is not clear, and 5 to 7 days was chosen arbitrarily based on similar data in patients after traumatic brain injury. All articles describe the use of central venous pressure or Swan Ganz catheter monitoring to determine fluid status followed by initial volume resuscitation with crystalloid and then colloid (whole blood or plasma) as indicated. If MAP remains below 85 mm Hg, most studies describe the use of pressors, typically a beta-agonist, followed by an alpha-agonist. Another recent review looked at the intensive care unit management of SCI, and recommended close attention to invasive hemodynamic monitoring and volume replacement or use of pressors as indicated .
Tator and colleagues studied 144 patients with SCI who were treated with close attention to the management of respiratory failure and aggressive treatment of hypotension with crystalloid and whole blood or plasma transfusion. They compared outcomes with a cohort of 358 patients who were treated at their center before institution of the above treatment principles. Overall morbidity and mortality were reduced, as were costs and length of stay; these improvements were attributed to the improved respiratory management and avoidance of hypotension.
Levi and colleagues treated 50 patients who had acute cervical SCI with a protocol that focused on improved cardiac output and keeping MAP >90 mm Hg by use of invasive monitoring along with volume and pressor support. Thirty-one patients were Frankel A, eight patients were Frankel B, and 11 patients were Frankel C and D. Eighty-two percent of patients had volume-resistant hypotension requiring pressor within the first 7 days of treatment, which was 5.5 times more common in those with motor complete injuries. These patients had a reduced peripheral vascular resistance index, with 58% having values below normal. Half the patients also had a reduced systemic vascular resistance index. The investigators noted that no patient with a motor complete injury and marked deficits in these vascular indices experienced neurologic recovery at 6 weeks.
Vale and colleagues managed a cohort of 77 patients with acute SCI with invasive monitoring and blood pressure support to maintain a MAP >85 mm Hg for 7 days after injury. The average pretreatment MAP for the American Spinal Injury Association (ASIA) A cervical patient was 66 mm Hg. Nine of ten cervical ASIA A patients required pressor to meet the MAP goal, and 52% of the incomplete cervical injuries needed pressors. Only nine of 29 thoracic level patients needed pressors to meet the MAP goal of >85. Minimal morbidity was associated with the use of invasive monitoring or pressors. Reported outcomes were very good, with three of ten cervical ASIA A patients regaining ambulatory function, and 23 of 25 patients with incomplete cervical SCI were ambulatory at 1 year. The investigators reported improved neurologic outcomes based on this strategy, but without a control group it is difficult to tell.
Ball discusses treatment of acute hypotension, which is caused by loss of vasomotor tone and pooling of blood in the peripheral and splanchnic vasculature. They recommend volume resuscitation of 1 to 2 L, with caution not to infuse excess volume in a normovolemic patient. Because of the interruption of the sympathetic cardioaccelerator fibers, the heart cannot compensate for the increased venous return with an increase in heart rate, thus, the only change that can occur is an increase in stroke volume (which may not be attainable). Thus, use of a vasopressor with both alpha- and beta-adrenergic actions (such as dopamine or norepinephrine) is ideal, to give the heart the chronotropic support as well as counteracting the lack of sympathetic tone to the vessels. This prevents complications associated with volume overload, including pulmonary edema.
Zach and colleagues managed 117 patients with acute SCI, using central venous pressure monitoring and volume expansion to maintain blood pressure for 7 days. They reported that 62% of the patients with cervical injuries improved, with two patients improving by two grades and one patient by three grades. No patient with a cervical injury worsened, and 38% were unchanged. Patients who were admitted within 12 hours of injury were more likely to improve than those admitted later (after 48 hours). The study was limited by lack of a control or comparison group. The investigators concluded that early management and close attention to maintaining an acceptable blood pressure improved prognosis.
Wolf and colleagues combined aggressive medical and surgical management of patients with acute cervical bilateral facet dislocations. Treatment included monitoring and maintenance of MAP above 85 mm Hg for 5 days in combination with immediate closed or open reduction of the cervical spine. They report improvement in 21% of patients with complete and 62% of patients with incomplete cervical injuries. The investigators concluded that their management protocol improved outcomes after acute cervical SCI. The study was limited by lack of a control group.
Bradycardia
Bradycardia often is seen in patients with cervical spinal cord injury because of a preponderance of vagal tone and a lack of descending sympathetic input. This bradycardia lasts for 2 to 6 weeks after injury; however, episodes of persistent bradycardia after that time may occur in severe injuries . Lehmann and colleagues studied 71 consecutive patients after SCI. All 31 with severe cervical SCI (Frankel A and B) had persistent bradycardia (<60 beats per minute). The severe cervical group also had more marked bradycardia (pulse <45); hypotension with systolic blood pressure of <90 mm Hg; supraventricular arrhythmias, predominantly atrial fibrillation; and primary cardiac arrest (16% versus 0 versus 0%). Twenty-nine percent of the severe cervical group required repeated injections of atropine or a transvenous pacer, whereas none in the other groups did.
In Lehmann and colleagues’ study, primary cardiac arrest occurred in five of 31 patients, and all were Frankel A. All had shown some cardiovascular abnormality, including hypotension and persistent bradycardia in all five, a need for pressor therapy in four, a need for atropine in three, and prior tachyarrhythmias and new AV block in two. Three of the cardiac arrests had a fatal outcome. The frequency of bradyarrhythmias peaked on day 4 after injury and subsided over the first 10 days. All observed abnormalities (including hypotension and bradycardia) resolved within 2 to 6 weeks. All these events are thought to be secondary to lack of sympathetic innervation to the heart in patients with cervical SCI. Evidence to support this includes the frequency of bradyarrhythmias in cervical SCI, low baseline serum catecholamines, a subnormal increase in heart rate with atropine, the prevention of bradyarrhythmias with low-dose sympathomimetics, and the ability of unopposed vagal tone to cause bradycardia, sinus arrest, AV block, and atrial fibrillation.
Severe bradycardia and sinus arrest have been reported to occur after vagal stimulation such as tracheal suctioning in tetraplegics . Hypoxia stimulates a sympathetic response through the pulmonary inflation reflex and a vagal response through carotid body chemoreceptor activation . Although in non–cord-injured individuals the sympathetic response dominates and produces tachycardia in the presence of hypoxia, in persons with cervical spinal cord injuries, the response to hypoxia is paradoxical bradycardia. This is thought to be caused by the lack of sympathetic tone that would normally counteract the rise in vagal tone with suctioning or other maneuvers (including defecation or even turning). The treatment of bradyarrhythmias with atropine is only partially and transiently effective, which is consistent with the lack of sympathetic tone as the cause rather than excessive parasympathetic tone. Use of low-dose isoproterenol was suggested by Lehmann and colleagues , which reportedly eliminated the sinus pauses.
The guidelines for implantation of permanent pacemakers in individuals with cervical spinal cord injuries and persistent cardiovascular abnormalities are not clear, and the literature in this area is limited to case reports. Ruiz-Arango and colleagues reported three cases of patients with cervical SCI who required permanent pacemaker implantation. All cases were patients with injuries at C5 or above who had recurrent desaturation and prolonged mechanical ventilation, episodes of hypoxia leading to profound bradycardia and asystole, and cardiac arrhythmias requiring temporary pacing. In this case series, the most common arrhythmia was bradycardia, but patients also had AV block (first-degree and Mobitz II), atrial fibrillation, sinus arrest with sinus pauses, and asystole. In two of these patients, vagal stimulation triggered asystole necessitating resuscitation. Bilello reported on 83 patients with tetraplegia and divided them into the high cervical group (C1 to C5) and the low cervical group (C6 to C7) . All patients had hypotension (systolic blood pressure <90) and bradycardia (heart rate <50), but 24% of the high group needed cardiovascular interventions including pressors, chronotropes, and cardiac pacing. Only 5% of the low group required cardiovascular interventions. Two patients in the high cervical group required permanent pacemakers.
Respiratory impairments
In addition to the cardiovascular consequences of spinal cord injury, which include hypotension and bradycardia, there are also pulmonary consequences of the loss of supraspinal sympathetic control. These cause respiratory impairments in addition to those created by the lack of innervation to respiratory musculature. The pulmonary vascular bed also has sympathetic innervation, and the lack of sympathetically mediated bronchodilation may exacerbate respiratory difficulties. In addition, neurogenic pulmonary edema occurs not uncommonly after acute SCI.
Neurogenic pulmonary edema is attributed to an immediate increase in blood pressure after SCI, with resultant increase in systemic and pulmonary vascular pressures. This increase results in a shift of blood from the high-resistance systemic circulation to the low-resistance pulmonary circulation . In addition, aggressive fluid resuscitation is not uncommon because of the inability to gauge fluid status in an acutely injured patient with severe hypotension; thus, the patient may be hypervolemic . Marked increases in pulmonary vascular pressures and in pulmonary blood volume then produce pulmonary edema because of the hydrostatic effect of increased pulmonary capillary pressure . In addition, pulmonary hypertension and hypervolemia injure pulmonary blood vessels, altering pulmonary capillary permeability. After the transient systemic and pulmonary vascular hypertension subsides, the patient is left with abnormal pulmonary capillary permeability, so that pulmonary edema persists . Neurogenic pulmonary edema has been reported to occur with autonomic dysreflexia, which supports the theory that a massive sympathetic discharge is the initiating event in neurogenic pulmonary edema .
The altered response of the pulmonary vascular bed after cervical SCI was studied by Levi and colleagues . These investigators treated 50 patients with acute cervical SCI and noted that patients with a low pulmonary vascular resistance index (PVRI) were less likely to recover any neurologic function than patients with a higher pulmonary or systemic vascular resistance index. They also noted that these patients have a reduced response to conventional volume challenge within the pulmonary vasculature, which is improved with small amounts of dopamine. They hypothesized that the poor prognosis for patients with low PVRI is reflective of the severity of the SCI and that the pulmonary vascular bed is more sensitive to the sympathectomized effects of cervical SCI.
Schilero and colleagues found that subjects with tetraplegia had significantly reduced airway conductance, which increased significantly after inhaled ipratropium bromide . They hypothesized that the reduced baseline airway caliber was due to heightened vagomotor airway tone, due to interruption of sympathetic innervation to the lungs. The anticholinergic properties of the ipratropium act to decrease vagal tone to the airways and allow bronchodilation.
Postacute cardiovascular responses
Neuronal activity in the spinal cord neurons slowly returns after SCI, marking the emergence from spinal shock . Spinal shock typically persists for 4 to 6 weeks, but there is still a lack of clarity regarding the definition of the end of spinal shock, because different reflexes tend to recover at different times. For example, the bulbocavernosus reflex returns within 24 to 48 hours after SCI, whereas deep tendon reflexes take 2 weeks to return after injury . Several studies have noted the return of heart rate and blood pressure to near normal values within 5 to 7 days of lower cervical and upper thoracic SCI . However, after the acute phase of SCI, alterations in cardiovascular function continue to exist because of the lack of central regulation of the autonomic nervous system.
In general, basal systolic and diastolic blood pressure in tetraplegics is about 15 mm Hg lower than that in normal subjects . This is because of the interruption of supraspinal sympathetic input and is reflected by low levels of plasma noradrenaline and adrenaline. As would be expected, an inverse relationship between level of injury and blood pressure has been found, which is thought to be the result of the lack of sympathetic vasoconstrictor influences below the level of injury . In persons with chronic tetraplegia, supine blood pressure is lower than in non–cord-injured persons with loss of the nocturnal circadian fall of blood pressure . Krum and colleagues studied 10 persons with cervical spinal cord injuries compared with 10 immobilized controls, measuring blood pressure and heart rate variability over a 24-hour period. Nighttime blood pressures were the same in both groups, but daytime blood pressures were higher in non–cord-injured persons. These variations were not associated with postural changes. The findings suggest that the normal diurnal variation in sympathetic activity is absent in persons with tetraplegia.
Other cardiovascular alterations after SCI include changes in heart and cardiac output. Individuals with mid-thoracic cord injuries have elevated heart rates at rest and with activity. In addition, they have lower stroke volumes at rest and with activity than non–cord-injured people . This elevated heart rate may be a compensatory mechanism for the reduced stroke volume, which is caused by decreased venous return from regions below the level of injury. This reduction in venous return is caused by both lack of effective muscle pumping action and lack of sympathetic vasoconstrictor tone .
In persons with chronic SCI, vascular adaptations are seen, including 30% reduction in femoral artery diameter, with a reduction in blood flow and doubling of stress levels in the femoral artery. These adaptations occur within the first 6 weeks after injury, with extensive reductions in femoral diameter and leg volume and increased basal shear rate levels and flow-mediated dilation . The change in limb volume caused by muscle atrophy parallels the time course of the arterial changes and is proportional, suggesting a functional link between muscular and arterial adaptations.
de Groot and colleagues found that the dimensions of the left ventricle, left atrium, and vena cava are all reduced in persons with cervical SCI. However, systolic and diastolic functions are not altered, suggesting that the changes in the cardiac structure are adaptive. These changes are hypothesized to be caused by a variety of factors including vascular atrophy and reduced total blood volume, reduced cardiac filling, stroke volume and cardiac output, and decreased preload caused by pooling of blood in the lower extremities, low systolic blood pressure, and lower heart rate. These may result in lower left ventricular (LV) wall stress and subsequent cardiac atrophy. Nash found that LV myocardial atrophy is common in persons with chronic tetraplegia but that exercise could cause remodeling and an increase in LV mass .
Changes in circulating catecholamines
Free plasma catecholamine measurements are used to assess current sympathetic nervous system activity because of their short half-life. Plasma noradrenaline levels reflect sympathetic nerve functioning, whereas plasma adrenaline levels reflect adrenomedullary function . Individuals with chronic tetraplegia have low basal levels of plasma noradrenaline and adrenaline . Those individuals with injuries between T1 and T5 also have low levels of adrenaline, indicating impaired release of catecholamines from the adrenal medulla . Individuals with injuries below T5 actually have levels of plasma catecholamines that are higher than non–cord-injured persons . With exercise, persons with cervical-level injuries do not have augmentation of catecholamines, those with lesions from T1 to T5 have increased noradrenaline but not adrenaline, and those with lesions below T5 have an increase in both catecholamines . This fact is again consistent with the known spinal level of innervation of the sympathetic outflow to the adrenal medulla.
Orthostatic hypotension
Blood pressure control depends on tonic activation of the sympathetic nervous system via descending input from supraspinal structures . In non–cord-injured persons, assumption of upright posture is associated with pooling of blood in the lower extremities, which reduces cardiac output. This causes a decline in blood pressure, which is sensed by the aortic and carotid sinus baroreceptors. This causes a decrease in the rate of inhibitory afferent action potentials to the medullary vasomotor center via the glossopharyngeal and vagus nerves . In response, there is sympathetic activation via descending spinal tracts, which causes vasoconstriction and also results in parasympathetic inhibition .
SCI interrupts the descending sympathetic input from the rostroventrolateral medulla, which is excitatory in nature. Without this tonic input, there is low resting blood pressure, loss of blood pressure autoregulation, and disturbed reflex control . Reduced blood volume in the intrathoracic veins leads to decreased venous return, decreased ventricular end-diastolic filling pressure and stroke volume, and decreased cardiac output and arterial blood pressure . In persons with SCI above T6, there is loss of vasoconstriction in the splanchnic bed, which contributes to loss of blood pressure autoregulation. Even in patients injured below T6, there is still loss of reflex vasoconstriction in the skeletal muscle bed, which may lead to a degree of orthostatic hypotension . In addition, persons with paralysis of the lower extremity musculature loose the beneficial effects of the venous pumping action of active muscle contraction, thus contributing to venous pooling. All of these changes contribute to orthostatic hypotension after SCI.
Orthostatic hypotension is a decrease in systolic blood pressure of more than 20 mm Hg or a decrease in diastolic blood pressure of more than 10 mm Hg with upright posture or head-up tilt to 60° for at least 3 minutes . This hypotension is typically accompanied by symptoms that are not necessarily related to absolute blood pressure. Symptoms of hypotension include fatigue or weakness, dizziness, light-headedness, blurred vision, dyspnea, and nausea . In one study, 41% of cord-injured persons with orthostatic hypotension were asymptomatic, but 74% of spinal cord injured persons had orthostasis . Orthostasis is severe acutely but persists for some patients chronically and may in fact worsen many years after injury .
Persons with spinal cord injury tolerate orthostasis well, perhaps because of changes in cerebral autoregulation that maintain cerebral blood flow and oxygenation despite greater decrease in MAP and stroke volume than non-cord injured . Typically, there is not a loss of consciousness with orthostasis, except in recently injured persons with tetraplegia or those with chronic tetraplegia after a period of recumbency. Studies in persons with tetraplegia have discovered the ability to autoregulate cerebral perfusion at lower systemic blood pressures. In the baboon model, cervical sympathectomy allows cerebral artery vasodilation and regulation of cerebral perfusion pressure at lower blood pressure levels than before sympathectomy.
There is a slight increase in heart rate during head-up tilt because of decreased baroreceptor activity causing a reduction in vagal tone. However, the heart rate does not typically go above 100 beats per minute because of lack of an intact sympathetic nervous system . This effect is different from that in non–cord-injured individuals, who may become quite tachycardic with hypotensive episodes. Plasma noradrenaline levels do not increase with head-up tilt as expected because of the lack of ability to activate the sympathetic nervous system reflexively in response to a fall in blood pressure. However, there are a variety of other mechanisms that help the person with tetraplegia adapt to orthostatic challenges. The release of renin occurs independently of sympathetic stimulation, probably secondary to the decrease in renal perfusion pressure . This results in the formation of angiotensin II, which is not only a vasoconstrictor but also facilitates peripheral NA release and the release of aldosterone from the adrenal cortex. Aldosterone serves to retain sodium and water, which increases intravascular volume . Vasopressin (antidiuretic hormone) also is released, which causes fluid retention and decreased urine output during prolonged head-up tilt.
The return of lower extremity spasticity can counter the lower extremity venous pooling and reduces the incidence of orthostasis in those with chronic SCI. Other mechanisms that decrease the degree of orthostatic hypotension experienced by persons with chronic SCI include vigorous renal vasoconstriction and some autonomy of spinal vasomotor reflexes . The management of orthostasis is discussed in detail in the review by Claydon and colleagues .
Temperature regulation
Although central temperature mechanisms are unaffected by spinal cord injury, there is impairment in the ability to regulate body temperature because of the loss of hypothalamic thermoregulatory control below the level of the injury. In addition, there is interruption of the afferent pathways from the peripheral temperature receptors below the level of SCI. In persons with spinal cord injury above T6, there is a complete loss of shivering, thermoregulatory sweating, and no peripheral circulatory adjustment below the lesion. Spinal cord–injured individuals have lower core temperatures in the cold than non-SCI individuals, resulting in the term partial poikilotherms . There is some evidence of spinal reflex–mediated sweating, but this remains a matter of debate. Wallin and colleagues found that changes in ambient temperature did not change the firing rate of sympathetic neurons, thus, leading to the conclusion that sympathetic thermoregulatory reflexes do not occur at the spinal level in humans. In paraplegics, deep or central temperature receptors sensitive to cold are able to initiate shivering above the level of the SCI. These receptors can act independently of the temperature of the skin above the SCI .
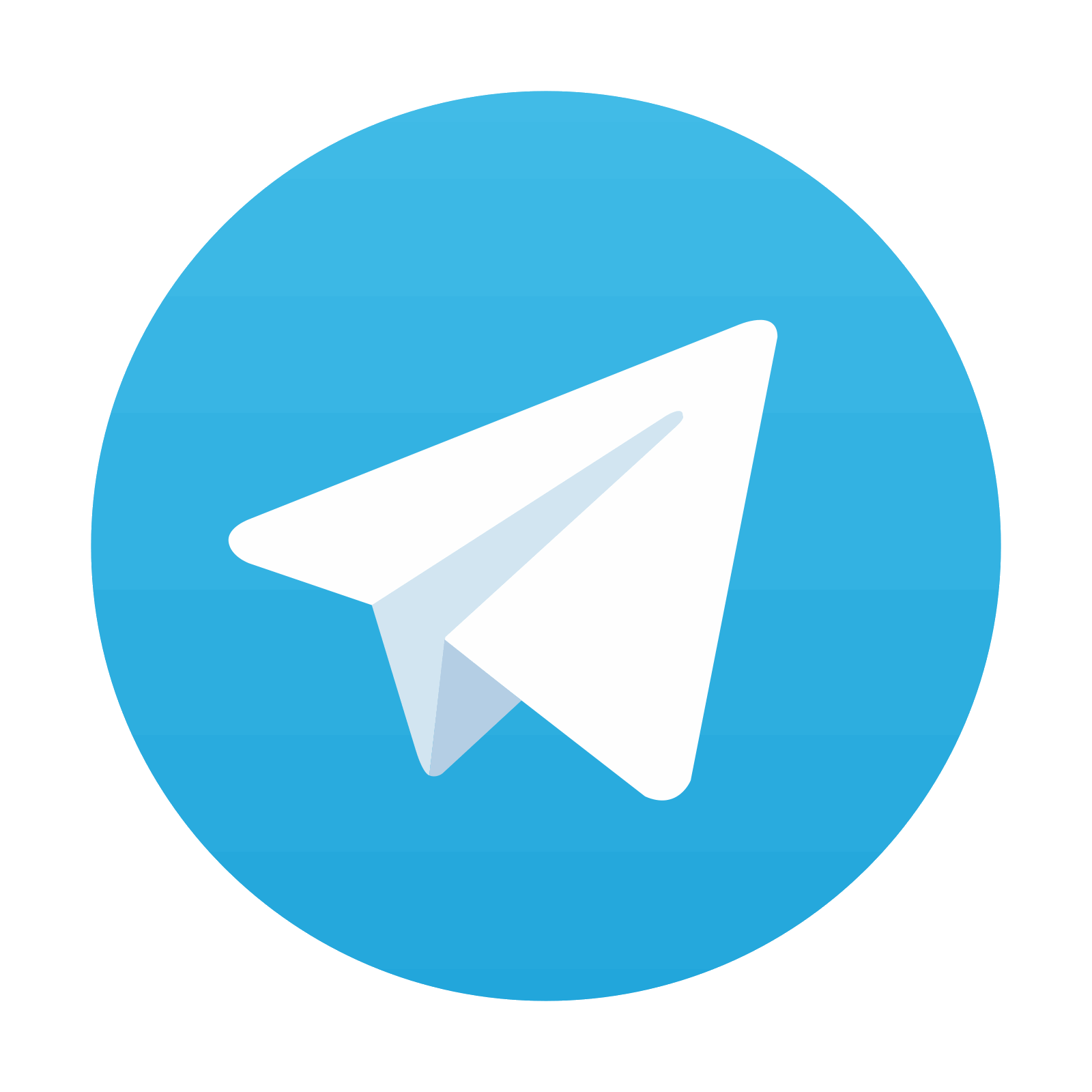
Stay updated, free articles. Join our Telegram channel
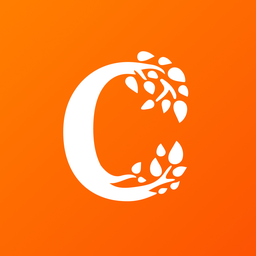
Full access? Get Clinical Tree
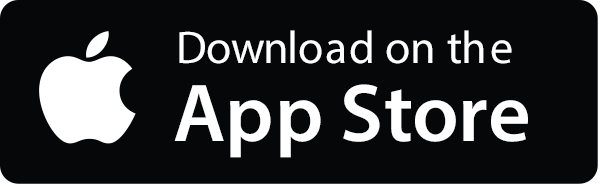
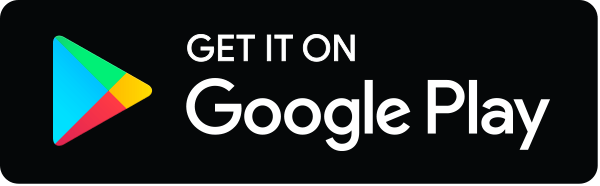