Abstract
Allograft tissues are commonly used by orthopedic surgeons and are processed using a variety of technologies to increase safety and clinical use. For safety, although disease transmission is a tangible risk, this possibility has been dramatically minimized through modern tissue-processing methods. These include steps to prevent processing tissues with unacceptable bioburden through rigorous screening using donor medical and social histories along with microbial testing of recovered tissue and viral testing of donor serum. Potential bioburden is also controlled through aseptic recovery and processing methods and then reduced through disinfection steps that can include antibiotics, detergents, mechanical process, chemical solutions, and terminal sterilization. Processing steps may also include decellularization methods to lower immunogenic potential of some tissues. To enhance fusion potential of bone void fillers, demineralization steps may be used, and the resultant demineralized bone matrices may be combined with a carrier to improve handling. Bone void fillers and osteochondral allografts may also be specially processed to retain a living cellular component. To preserve relevant biological, biochemical, and physical properties of allografts for clinical use and ease of handling, a number of methods may be used which include: (1) refrigeration in media, (2) freeze-drying, (3) cryopreservation, (4) freezing, and (5) media storage at room temperature. As academic and industry research continue to drive advances, the future direction of allograft tissue likely includes injectables, coatings, cellular therapies, and combinations with other materials. The technology approaches outlined here will be further described along with future directions.
Keywords
Allograft, Bioburden reduction, Bone void fillers, Decellularization, Disease transmission, Gamma irradiation, Preservation
Disclosure Statement
Dr. Moore, Mr. Samsell, and Dr. McLean are employees of LifeNet Health, a nonprofit organization.
Introduction
Biologic tissues are among the many clinical options available to orthopedic surgeons, with over 1 million annual implants of human allografts alone. Such biologic tissues may be considered structural, for example, tendons or cortical bone struts, or nonstructural, such as ground demineralized bone matrices (DBMs) or amniotic membranes. Their intended use may mimic their original anatomical function, such as using a patellar ligament for anterior cruciate ligament (ACL) reconstruction or a cortical bone segment to repair a long-bone fracture. In other circumstances, their use may differ from original anatomy, such as using a dermal layer for rotator cuff repair or a ground amniotic membrane combined with demineralized cortical bone for a spine fusion procedure. This chapter focuses on the intent and science of various means of processing human allograft tissues to ensure safety and clinical use.
Although xenografts, derived from nonhuman sources, have found clinical use in a variety of surgical disciplines, including the use of porcine heart valves for heart valve replacement surgery and ground bovine bone for dental procedures, their use in orthopedic surgery has met with more limited use and success. In particular, porcine-derived small intestinal submucosa did not perform well as a tissue augmentation material, and bovine tendons are not commonly used ; thus, xenografts will not be discussed in this chapter.
Although autografts, taken directly from the patient, are widely used for procedures such as ACL reconstruction or spinal implant of an iliac crest segment, these grafts are not subject to any significant processing other than some cleaning, shaping, or suture attachment before intrasurgery transplantation and therefore are also not covered in this chapter. Other autograft materials, such as those used for autologous cartilage implantation or blood-derived preparations such as platelet-rich plasma, are described elsewhere in this book.
Before being used by the orthopedic surgeon, allografts are commonly processed through physical, chemical, or biochemical means. Such processing steps are typically performed to accomplish one or more objectives such as:
- •
to reduce risk of disease transmission (e.g., through various disinfection or sterilization steps);
- •
to reduce immunogenic response (e.g., through decellularization);
- •
to reduce barriers to optimal physiological activity (e.g., by demineralizing cortical bone to enhance bioavailability of growth factors);
- •
to physically convert grafts into more usable forms (e.g., shaping a bone graft for placement as an intervertebral body spacer);
- •
to combine grafts with synthetics to enhance ease of use (e.g., by combining ground demineralized bone with a carrier to produce a putty-like material);
- •
to preserve tissue to increase shelf life or simplify storage (e.g., lyophilization of ground bone that enables retention at ambient temperatures).
With the focus here on human allografts, a brief historical and regulatory perspective is provided as background, followed by sections on reduction of disease transmission, enhancing fusion potential of bone void fillers, lowering immunogenic response by decellularization, preservation methods, and future directions.
Background
Human allografts have been used in orthopedic surgery for many decades. In the 19th century William Macewen described the successful use of allogeneic cortical bone fragments to graft a replacement for a missing humeral mid-shaft. Over 100 years ago, Fred Albee published a long list of surgical uses of bone allografts including applications and stated, “ [I have] been able during the past 2 years to avoid entirely the use of metal … for internal bone fixation purposes … made possible, largely, by utilizing the best of well known mechanical devices hitherto rarely, if at all, used in surgery, such as bone inlays, wedges, dowels, tongue and groove joints, mortised and dove-tailed joints. ”
Through the 20th century bone grafts continued to be used, and tendon allografts started gaining widespread acceptance by the 1980s. During the mid- to late-20th century, most “bone banks” for allografts were hospital-based with tissue derived from deceased or amputated patients. These tissues often underwent only minimal chemical processing, such as antibiotic or disinfectant soaks. In addition, any physical alteration of these tissues was typically performed by the surgical team at the time of implantation and included bone shaping or grinding or tendon trimming or suturing. In answer to the need for better defined methods for ensuring graft safety and consistency, as well as appropriate respect for the tissue donors and their families, more formal systems began to be established by the Navy Tissue Bank in Bethesda, MD.
Tissue Bank Standards and Regulation
In the latter half of the 20th century, the methodologies and practices developed by the Navy Tissue Bank were increasingly adopted by other organizations. In 1976 the American Association of Tissue Banks (AATB) was formed, and in 1984 it issued the first set of tissue-processing standards, which established guidelines such as acceptable time from death to recovery, storage conditions for tissues, microbial testing requirements, definitions of demineralization, freeze-drying, and so forth. A certification program was also established to assure the surgical community that qualified tissue banks met AATB standards.
Similarly, around the turn of the 21st century, the US Food and Drug Administration (FDA) developed the classification of Human Cell and Tissue Products (HCT/Ps) as a separate regulatory classification into which most human tissue transplants fell. This classification applies to most human tissue transplants. It differs from a medical device classification, in which, to qualify as an HCT/P, the tissue needs to meet standards of not exceeding “minimal manipulation” such that the “original relevant characteristics” are not altered, and also “homologous use” meaning the tissue is used clinically in a similar manner to that intended in the body of origin. A clear example of a tissue meeting these requirements would be a hamstring tendon that is recovered intact, disinfected, sterilized, and then used for tendon replacement. Conversely, although bone void filler putty containing DBM would be considered for homologous use, it may be considered more than minimally manipulated because of the addition of a synthetic carrier and thus be classified as a medical device requiring FDA clearance before distribution. In further example, a disinfected and freeze-dried cortical bone segment used as an intervertebral body spacer is considered both minimally manipulated and intended for homologous use. As processors continue to become more innovative in the use and treatment of human tissues, these definitions will undoubtedly be tested and clarified.
In adding further regulatory safeguards especially with respect to the risk of disease transmission, FDA issued the “Interim Rule” in 1993 to “require certain infectious disease testing, donor screening, and recordkeeping facilities to help prevent the transmission of AIDS and hepatitis through human tissue used in transplantation.” In general, methods to reduce risk of disease transmission, such as antibiotic soaks, peroxide disinfection, and sterilization using radiation methods, are considered to be no more than minimal manipulation. Further on the regulatory front, in 2005, FDA established, in conjunction with tissue bank input, a standard of good tissue practices to “create a unified registration and listing system for establishments that manufacture HCT/Ps and to establish donor eligibility, current good tissue practice, and other procedures to prevent the introduction, transmission, and spread of communicable disease.”
In summary, the US system of AATB Standards and FDA regulations and inspections provide many safeguards for the provision of effective transplantable allograft tissues.
Reducing Risk of Disease Transmission in Transplanted Allografts
Human tissue carries an inherent, yet minimal, risk of disease transmission, which has been made essentially negligible through advancements in cleaning and processing methodologies. A 2005 survey from AATB estimated an overall allograft-associated infection rate of 0.014%. It is important to note that this survey preceded widespread implementation of more advanced methods aimed at reducing the risk of disease transmission, including FDA-mandated and sensitive nucleic acid testing (NAT) for certain viruses as well as routine terminal sterilization. Although well-documented cases of disease transmission have occurred, modern tissue bank practices and processes have successfully diminished incidences over the last few decades. In a case beginning in 1985, four organs and 54 allograft tissues were distributed from an HIV-infected donor who had a favorable screening history and negative serology test. All four organ recipients and the three recipients who received fresh frozen bone tissue tested positive for HIV. Investigators suspected that the transmissions occurred because the seronegative donor had very recently become infected and, being in the “window” period, had not yet developed an HIV-1 antibody detectable by the tests in use at the time. In 2002 another case surfaced where 40 recipients received organs and tissues from a donor infected with hepatitis C virus (HCV), and 8 consequently developed HCV infections. As with the earlier HIV transmission case, the donor was seronegative for HCV, and NAT had not been performed. Subsequent NAT of stored serum from the donor detected the virus, which highlighted the importance of using more sensitive testing before releasing donor tissue. NAT for HIV and HCV is now required by FDA after being added as a requirement by AATB in 2005. There have also been reported cases of transmission of tuberculosis, various Clostridium species , Group A Streptococci , and rabies. Overall, the relative risk of disease transmission is small considering the millions of allografts transplanted; however, the possibility of transmission makes avoidance, control, and reduction of microbial and viral bioburden integral to tissue-processing practices.
Reducing Risk of Disease Transmission: Approach
Allograft tissue providers reduce risk of disease transmission by three primary means:
- 1.
minimizing occurrence of processing donor tissue with unacceptable bioburden;
- 2.
controlling environment and tissue-handling practices to avoid contamination; and
- 3.
reducing any remaining bioburden through disinfection and sterilization techniques.
In the first category, the occurrence of recovering or processing tissue that is contaminated can be minimized through stringent bioburden tests, including anaerobic and aerobic culture tests for bacteria and fungi, as well as serological testing and NAT to detect specific viruses. Specific tests are required by FDA and also to meet AATB standards. Note that proper infectious disease tests must be validated for use specifically with cadaveric specimens. A detailed donor-screening process is also critical for minimizing bioburden; medical records and social history, such as travel, tattoos, high-risk sexual behavior, illicit drug use, and incarceration, as well as physical examination, help assess donor eligibility.
In the second category, bioburden loads can be controlled by using aseptic handling techniques during recovery and processing to prevent contamination of the tissue by pathogens. However, it is important to note that using aseptic conditions by themselves can only prevent additional contamination but will not reduce or eliminate any existing bioburden. For the third risk-reduction category, processing methods designed to reduce any remaining bioburden are addressed in the following sections.
Bioburden Reduction Methods
Bioburden loads can be reduced by cleaning and disinfecting the tissue. These steps vary by tissue type and may include:
- •
debridement;
- •
low doses of preirradiation (irradiation before other chemical processing steps);
- •
physical methods such as lavage, pulsatile fluid flow, centrifuge, fluid bath rotation, and sonication;
- •
enzymatic digestion of cellular material;
- •
penetrating agents such as supercritical CO 2 in combination with chemical activators;
- •
milder chemicals including alcohol, detergents, and antibiotics;
- •
more aggressive chemicals such as NaOH, acetone, and peroxide.
Cleaning processes can remove bone marrow elements, lipids, and low-molecular-weight proteins, thus reducing any graft immunogenic potential as well as bacterial, viral, and fungal contamination. More aggressive agents that may be commonly used to disinfect bone, such as hydrogen peroxide, are not typically used for soft tissue grafts. At least one process that includes the use of hydrogen peroxide on tendons was correlated with a significant increase in risk for revision ACL repair. In this case, however, the contribution of the hydrogen peroxide to apparent graft weakening is unclear because the process also includes pulsatile fluid flow. Cellular remnants and, presumably, associated infectious agents may also be removed through tissue decellularization methods, which will also be discussed in a later section. These methods include the use of chemicals such as nondenaturing anionic detergent, recombinant endonuclease, sodium dodecyl sulfate, sodium hydroxide, sodium peroxide, sodium chloride, and antibiotics.
Assurance of Tissue Sterility
After processing to reduce or eliminate bioburden, the tissue is typically either tested for sterility or subjected to a terminal sterilization step. Testing for sterility usually involves sampling a portion of the tissue or processing solutions according to United States Pharmacopeia <71> sterility test guidelines before packaging. It is important to note that the United States Pharmacopeia <71> label does not necessarily indicate that the tissue product is sterile but rather that a sample of the product batch was culture negative thus passing the test for sterility. As an alternative to testing for sterility, the tissue may be processed, packaged, and then subjected to sterilization after placement in its final package thus at the terminus of the process. In this case of terminal sterilization the chosen method must effectively penetrate the packaging; such options will be discussed in subsequent sections.
In terms of measuring sterility, an absolute assurance of sterility is not probabilistically feasible, but there is a measure known as sterility assurance level, commonly referred to as SAL. This designation indicates the degree, or level, of assurance of sterility achieved through a validated sterilization process. The two most common SALs for allografts are 10 –3 and 10 –6 . A product labeled with an SAL of 10 –3 indicates that there is a 1 in 1,000 chance of a single viable microbe present in the tissue, whereas an SAL of 10 –6 indicates a greater degree of sterility with a 1 in 1,000,000 chance of the same. An SAL of 10 –6 is the required level of sterilization by the Center for Disease Control and Prevention for medical instruments that breach the skin and is thus often referred to as a medical device level of sterility. This level of sterility can only be achieved through terminal sterilization, though some terminally sterilized tissue products still retain an SAL of only 10 –3 .
Note that SAL is only a measure of microbial sterility and is unrelated to viruses, although the methods used to achieve an acceptable SAL may be effective at lowering viral risk. As mentioned previously, tissue processors use strict donor-screening and viral test methods to prevent viral transmission. Furthermore, several processing methods have been demonstrated to result in significant viral inactivation. For example, low-dose gamma irradiation was demonstrated to inactivate a broad spectrum of viruses, including HIV and enveloped, nonenveloped, DNA, and RNA viruses. Another processing method uses vacuum and oscillating pressure combined with chemical agents to inactivate pathogens. The use of a terminal sterilization process with the ability to inactivate a wide range of virus types is particularly advantageous as new viruses (e.g., Zika, severe acute respiratory syndrome, and so forth) emerge for which validated blood tests may not yet exist.
Terminal Sterilization
Reported methods to terminally sterilize allografts in their final packaging include plasma H 2 O 2 , ethylene oxide (EO), supercritical CO 2 , electron beam irradiation, and gamma irradiation, all with benefits and risks. Plasma H 2 O 2 has the disadvantage of removing osteoinductive potential from DBM and may damage soft tissues. Although EO is commonly used to sterilize medical instruments, it has been reported to cause persistent synovial effusions and inflammatory responses in EO-treated allografts. Both supercritical CO 2 and electron beam irradiation have been reported capable of safely providing terminal sterilization without significantly damaging tissue. However, these processes are relatively unproven clinically and account for a small percentage of sterilized tissue. The most frequently used method of terminal sterilization is gamma irradiation.
Gamma Irradiation
Gamma irradiation is the most common sterilization treatment method used on allografts and is also used in a majority of processed tendons whether for terminal sterilization or as an intermediate step. Although there have been reports of negative outcomes with gamma-irradiated allografts, the tissues studied were either first treated with harsh chemical pretreatments or by applying irradiation at higher, less desirable temperatures. Other studies have demonstrated that the use of irradiated grafts did not negatively affect clinical efficacy.
In interpreting these inconsistent results, it is important to note four key variables that allow a reader to more accurately evaluate the method used for applying gamma irradiation and the potential impact on clinical outcomes. These four key variables include:
- •
target dose
- •
dose range.
- •
temperature at irradiation
- •
tissue treatment before irradiation
Target dose refers to the intended dose delivered to the tissue, although tissues actually receive a range of dosages due to the nature of irradiation. A dose range is a more precise description because it conveys both the minimum and maximum amount of radiation exposure a graft has received. A narrow dose range indicates a higher degree of control. Bone allografts irradiated with approximately 25 kGy (or 2.5 Mrad) have shown similar clinical results to nonirradiated bone, but irradiation dosages greater than this may negatively impact biomechanical properties, although the clinical significance is unclear. Soft tissue allografts are reported to perform well when treated at <20 kGy (2.0 Mrad).
The irradiation of allografts at low temperatures, e.g., on dry ice, has also been shown to effectively mitigate potential damage to the graft by minimizing the generation of free radicals. If the temperature of irradiation is not provided in a study, it may indicate that the grafts were irradiated at ambient temperature and more prone to damage. Thus, the temperature of irradiation should always be taken into account when analyzing the results of a study. Some studies report results that come from grafts irradiated at ambient temperature; it is important to note that these outcomes cannot be accurately applied to grafts more carefully irradiated at ultra-low temperatures. In support, after reviewing 5968 cases of ACL repair, Tejwani et al. did not find an increased risk of revision surgery with the use of two proprietary process that use low-dose (<1.8 Mrad) gamma irradiation applied at a low temperature. This outcome, along with the findings of other studies, support the clinical efficacy of low-temperature irradiated sterile tendons.
In summary, advanced tissue recovery and processing methods include steps to ensure bioburden prevention, control, and reduction. However, methods used by tissue processors vary at each step resulting in differences among available allografts. Tissue may either be offered aseptically or with a terminal sterilization step to reach an SAL of 10 –3 or 10 –6 . The type of terminal sterilization used also differs and can impact the clinical performance of the allograft tissue. Low-dose gamma irradiation at a low temperature is still the most common method with well-documented success, but bench-top studies have shown encouraging early results for supercritical CO 2 and electron beam irradiation.
Enhancing Fusion Potential of Allograft Bone Void Fillers
Bone allografts have long been used to provide structural support and to fill voids. Traditionally, these grafts have been processed as previously described to reduce risk of disease transmission and also to meet specific surgical dimensional needs through cutting, machining, and grinding. Traditional allografts, such as cortical struts, ground cortical bone, cancellous cubes, femoral heads, and shaped interbody spacers, have played, and continue to play, a prominent role in orthopedic surgery. In addition, as reviewed in this chapter, when accelerated fusion is an objective, the orthopedic surgeon now also has a wide array of more advanced options to facilitate new bone growth.
The breakthrough in this field came with the discovery that certain extractable bone elements could promote bone growth and, subsequently, when Marshall Urist and colleagues isolated the proteinaceous factors that were found to stimulate or induce new bone formation. This family of osteoinductive proteins was named bone morphogenic proteins (BMPs).
We now recognize that three key properties are required for new bone formation and growth: (1) osteoconductivity (scaffolding), (2) osteoinductivity (signals such as BMPs), and (3) osteogenicity (cells). In order, new bone must have a scaffold to grow, referred to as an osteoconductive matrix. Then cytokine signals are required to induce precursor cells to either differentiate toward the osteoblastic lineage or for cells to further express the osteoblastic phenotype of bone formation; these factors are thus considered osteoinductive. Finally, new bone growth requires the presence of bone-forming cells such as osteoblasts or precursors to produce an extracellular, mineralizing matrix, and this cellular component is referred to as osteogenic. Different bone-grafting options may provide one, two, or all three of these properties. For example, a processed cancellous cube is still considered an osteoconductive scaffold capable of supporting new bone growth by interacting with host bone and signals despite potentially having neither significant osteoinductive capacity, being low in native growth factors, nor any osteogenic potential, being devitalized. In another example, a recombinant form of one commercially available specific human growth factor, BMP-2, would be considered as an osteoinductive signal but would require the additional presence of osteogenic and osteoconductive components to drive bone formation. Furthermore, an autograft bone and marrow mixture derived from the patient may theoretically provide all three components necessary for new bone formation, but the quantity and quality of grafting material may depend on the surgical site, health, and age of the patient. As an alternative, viable cellular allografts can theoretically provide all three components, and these will be described further in the following sections.
Beyond simply filling bone voids, allografts can also be processed in different manners to enhance fusion potential. The most prevalent processing method involves taking advantage of the pervasiveness of native BMPs found in cortical bone. Through careful demineralization, these factors can become bioavailable in the resultant DBM. Typically, a dilute hydrochloric acid solution is used to dissolve some of the bone’s mineral phase (apatitic calcium phosphate), thus exposing these growth factors. In fact, this process mimics the natural action of osteoclasts in bone remodeling as they create a localized acidic environment which similarly dissolves the mineral phase, releasing BMPs to signal cells to lay down new bone. If undertreated, hypodemineralization would result in osteoinductive factors still being trapped in the bone matrix and unavailable for rapid signaling, whereas hyperdemineralization may lead to those factors either being eluted out of the matrix during demineralization or overly acid-exposed and denatured, thus yielding a nonosteoinductive material.
At least one study demonstrates this relationship by correlation of the residual calcium levels, as a measure of demineralization, to new bone formation using a rodent bone growth model. The authors support the premise that overdemineralization or underdemineralization lowers the osteoinductive potential of DBMs, indicating that there is an optimal range in the middle. Thus given the presence of bioavailable growth factors within a human bony matrix, properly demineralized DBMs are considered both osteoinductive and osteoconductive, still relying on the patient’s own cells for the osteogenic component. To improve handling, many DBM formulations also use a carrier such as glycerol, hyaluronic acid, starch, and so forth. An extensive study of the impact of these carriers on long-term clinical outcomes is generally lacking, although clinical evidence supports glycerol and hyaluronic acid as DBM carriers.
More recently, cellular bone allografts have emerged as a new option. These grafts are formulated to provide all three elements of bone formation. The osteoconductive component is bone in the form of chips, fibers, or particulates. The osteogenic component is comprised of cells either adhering to the bone component or added from another source, for example, adipose-derived cells or amniotic tissue. The osteoinductive component may be DBM derived from the same donor or may rely on endogenous trophic factors inherent to the cellular component. Most current cellular bone void filler approaches rely on inclusion of mesenchymal stem cells, assuming that they will produce factors conducive to the healing process and that they will differentiate down the osteoblastic lineage to initiate bone formation. The impact of the patient’s local environment on the differentiation pathway and timing is not well understood. This question becomes increasingly complex when the cell component derives from a nonbone source such as placenta or adipose tissue. Alternatively, cellular bone void fillers can include living cells that are integral to the donor bone and thus, being “bone cells,” are already committed to the osteoblastic lineage. Longer term, controlled clinical data would be beneficial in helping support providing the orthopedist with a single grafting material with all three components of bone formation.
Lowering Allograft Immunogenic Potential: Decellularization
Most allografts are generally considered nonimmunogenic either due to the nature of human tissue being transplanted into humans, as a consequence of cleaning processes that remove cellular material such as bone marrow elements, or through preservation processes, including freezing and freeze-drying. However, depending on the tissue type and intended clinical application, unprocessed allograft tissue can pose issues with immunogenicity, which need to be minimized for an allograft to be optimally biocompatible upon implantation. Furthermore, different types of tissue exhibit varying degrees of immunogenicity, as measured by antigenicity. Skin is generally more immunogenic than bone and tendon tissues, which exhibit lower immunogenicity that can generally be resolved through the typical allograft-cleaning processes. More immunogenic tissues may need alternative processes to lower antigenicity. One method treats tissue with glutaraldehyde, an aldehyde fixative, to greatly reduce immunogenicity by cross-linking antigens. However, glutaraldehyde has been associated with allergic reactions and may have contributed to the extremely high ACL failure rates reported in one study. Another method, frequently applied to dermal tissue, uses decellularization to reduce potential immunogenicity and is intended to yield a biocompatible scaffold as a favorable environment for host recellularization and remodeling. Although there are several different decellularization methods in use, the common goal is to eliminate cellular remnants that could cause an immune response while preserving the tissue architecture and maintaining the mechanical properties of the scaffold. Once the dermal tissue is decellularized, this scaffold can be used in orthopedic procedures, such as superior capsule shoulder reconstruction, Achilles tendon repair, and others.
As noted previously, decellularization processes can include both chemical and mechanical extraction methods. More specifically, decellularization techniques are reported to use anionic agents (e.g., sodium dodecyl sulfate, sodium chloride), alkali compounds (e.g., sodium hydroxide), and oxidizing agents (e.g., hydrogen peroxide) to solubilize cellular remnants before removal. However, the type of chemicals used and the duration of the decellularization process can affect the strength of the graft by removing collagen and glycosaminoglycans in addition to the immunogenic components, making the differences among decellularization processes worthy of consideration.
The amount of cellular remnants remaining after decellularization may be indicated using residual DNA content; a lower DNA content would theoretically indicate a cleaner matrix and more favorable host response. The residual DNA content of grafts varies widely. Published reports include ranges from less than 25 to greater than 250-ng DNA/mg dry weight for acellular dermal matrix (ADM) tissues. As residual DNA levels may correlate with host response, it is not surprising that different ADMs incorporate at different rates, as specifically noted when an ADM with lower residual DNA content was found to more rapidly integrate and recellularize in a comparative animal model.
Recellularization
Once an ADM has been implanted, host cells may begin repopulating the scaffold and initiating incorporation of the graft. This process starts with the migration of inflammatory host cells, followed by matrix remodeling, and then finally revascularization, leading to recellularization. Although the exact mechanism of host integration is not fully understood, differences in incorporation rates likely begin at the initiation of the process. The initial inflammatory host response is mediated by macrophages and monocytes, which must achieve a careful balance between wound healing and tissue destruction. Macrophages regulate the expression of interleukin-1b, a cytokine which contributes to the wound-healing process by controlling fibroblast activation. The M1 macrophage phenotype is more associated with an inflammatory response, whereas the M2 macrophage phenotype is associated with tissue repair and constructive tissue remodeling. Agrawal et al. investigated the mechanism of incorporation by examining the effect of macrophage phenotype expression on matrix remodeling using three different human ADMs and one synthetic/bovine ADM. The ADM products displayed differing patterns and timing of macrophage infiltration, leading the authors to conclude that this variability was due to the distinct decellularization processes used for each of the ADM products. Capito et al. also concluded that different decellularization processes may have accounted for the varying degrees of cellular and vascular ingrowth shown by the four different materials in this study.
As discussed in this chapter, decellularization can be beneficial for further reducing immunogenicity of certain tissue types. Different methods can result in different rates of recellularization, which may impact incorporation rates and ultimate tissue remodeling.
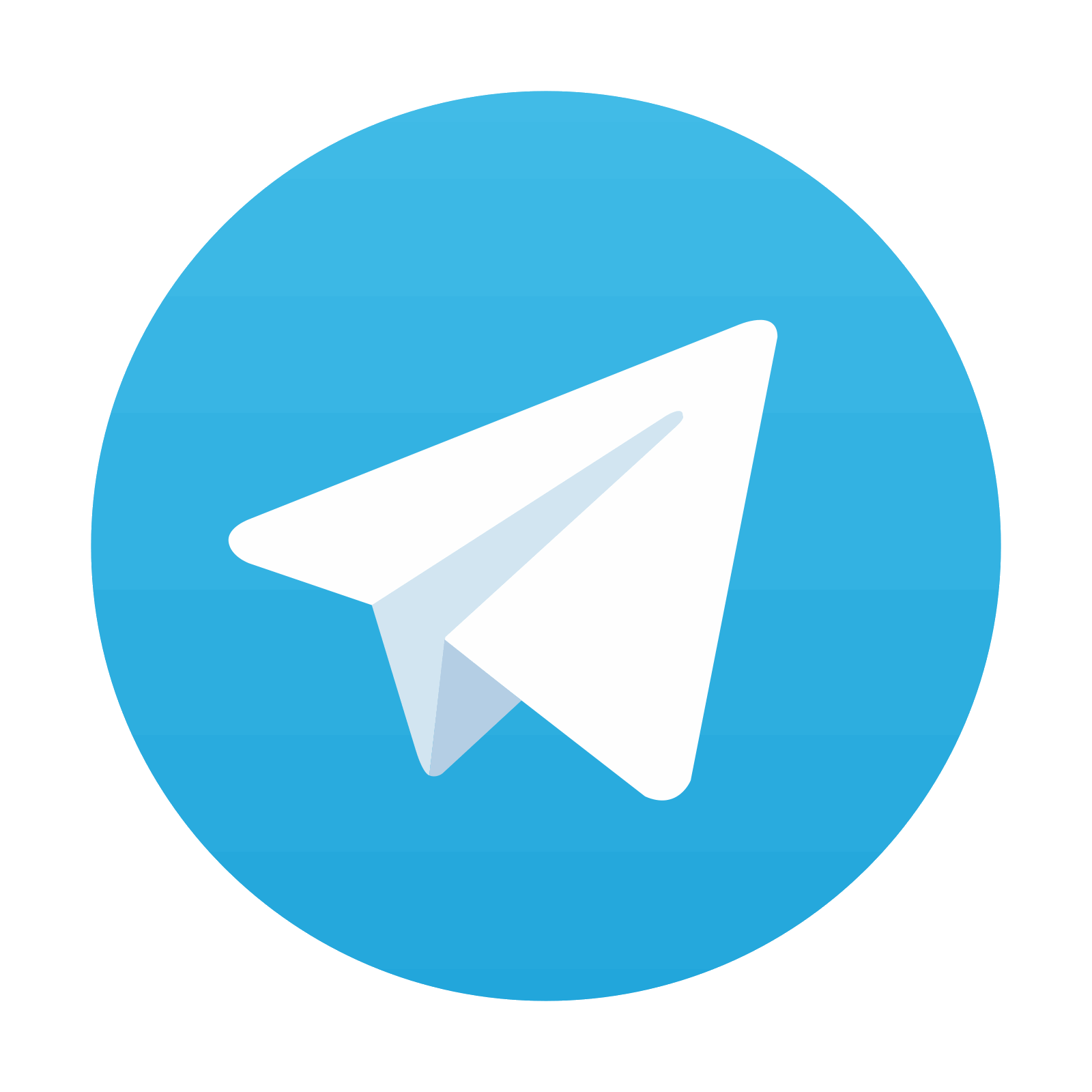
Stay updated, free articles. Join our Telegram channel
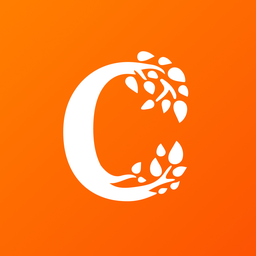
Full access? Get Clinical Tree
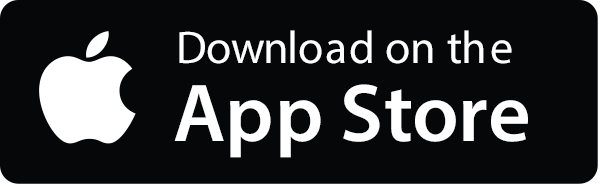
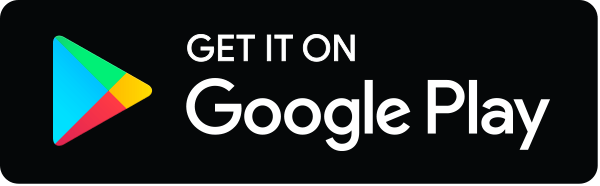