Fig. 8.1
(a) Radiograph of a failed total knee arthroplasty, demonstrating medial tibial collapse with component liftoff on the lateral side. (b) This knee was reconstructed using a custom tibial component. (c) Radiograph of a failed total knee arthroplasty with similar failure mechanism. (d) Tibia was able to be reconstructed with use of a metaphyseal sleeve and long uncemented stem

Fig. 8.2
(a) Radiograph of a failed total knee arthroplasty, demonstrating femoral subsidence worse on the medial side. (b) Femoral head allografts were used to reconstruct the extensive bone loss and reestablish the joint line in this 2-year postoperative radiograph
Although less commonly reported than in total hip arthroplasty, osteolysis from polyethylene debris does occur in cemented and cementless total knee arthroplasties [7]. High contact stresses secondary to poor design or technique can result in large volumes of debris. Regardless of particle size, large particle volume can cause early failure and catastrophic bone loss that is almost always underestimated by plain radiographs. As implant design, technique, and polyethylene quality have improved longevity, there is speculation that osteolysis may become a more common cause of late failure as well [8]. The surgeon should be prepared for major bone loss in a patient with a loose, painful total knee and any hint of cystic changes on X-ray.
Poor implant removal technique at the time of revision surgery is a further cause of bone loss. Patience is the key to removing any implant, well fixed or otherwise. It is imperative for the surgeon to expose the implant-bone interface. In cemented implants, disruption must occur at the implant-cement interface, not the cement-bone interface. Thin osteotomes are useful in this regard; however, one must fight the temptation to lever the implant out, as this may crush the underlying soft cancellous bone. Well-fixed cementless implants are difficult to remove. The use of a Gigli saw beneath the anterior flange and along the posterior condyles coupled with thin curved osteotomes in the notch is recommended. The use of slap hammers should be avoided until complete implant loosening is confirmed. Ill-advised use of these devices can easily lead to femoral or tibial fractures. In some instances, a condyle or entire distal femur may be removed with the well-fixed implant [9].
Defect Classification
Assessment of bone deficiency is best done after implant removal and preliminary cuts; however, the surgeon must have a reasonable expectation of the type of bone loss from preoperative X-rays. Several classification systems have been developed in the hip to assist revision surgeons. There has been less emphasis on defect classification in the knee. As in the hip, defects are generally divided into contained or segmental . Contained defects are surrounded by intact bone, whereas segmental defects have no remaining cortex [10]. Segmental defects can be further broken down into circumferential or non-circumferential [9]. Engh and Rubash have both devised classification schemes that attempt to correlate type and severity of defect with a recommended surgical management strategy (Tables 8.1 and 8.2) [5, 9]. Placement into one of these classification systems preoperatively allows the surgeon to have appropriate instruments, hardware, and graft on hand to limit intraoperative surprises.
Table 8.1
Anderson Orthopaedic Research Institute bone defect classification guidelines
Preoperative radiographs | Surgical management | |
---|---|---|
Type 1 defect (intact metaphyseal bone) | A full metaphyseal segment | No augments, structural bone grafts or cement fill >1 cm |
Femur | Metaphyseal bone intact distal to the epicondyles | |
No component subsidence or osteolysis | ||
Tibia | Metaphyseal bone intact above tibial tubercle | |
No component subsidence or osteolysis | ||
Type 2 defect (damaged metaphyseal bone) | A shortened metaphyseal flare | Joint-line restoration with augments (>4 mm), particulate or chunk bone graft, or >1 cm cement fill; joint-line elevation with a primary component as the revision implant |
Femur | Component subsidence or joint-line elevation of the failed component | |
Tibia | Small osteolytic defects in bone distal to the epicondyles | |
Component subsidence or position up to or below the tip of the fibular head; a shortened tibial metaphyseal flare | ||
Type 3 defect (deficient metaphyseal bone) | A deficient metaphyseal segment | A reconstructed condyle or plateau with structural graft or cement or a custom or hinged component |
Femur | Bone damage to or above the level of the epicondyles | |
Component subsidence to the epicondyles | ||
Tibia | Bone damage or component subsidence to the tibial tubercle |
Table 8.2
Massachusetts General Hospital femoral defect classification system for total knee arthroplasty and treatment algorithm
Classification | ||
---|---|---|
Minor | Below the level of the epicondyles Volume <1 cm3 Contained: no cortical bone loss, cancellous defects only Uncontained: cortical loss resulting in an unsupported portion of the implant | |
Major | Defects are at or above the level of the epicondyles Volume >1 cm3 Contained: no cortical bone loss, cancellous defects only Uncontained: cortical loss resulting in an unsupported portion of the implant or condylar fracture | |
Treatment algorithm | ||
Defect type | Minor | Major |
Contained | Particulate graft Cement Implants: CR or PS +/− stem | Bulk allograft Femoral head allograft Implants: PS with stem, possible constrained condylar |
Uncontained | Augments Structural graft Cement or particulate graft if <5 mm fill and varus/valgus stable | Condylar allograft Bicondylar allograft |
Implants: PS with stem | Distal femoral allograft Implants: constrained condylar with long stem or hinged device |
Several options for each category of deficiency are available to the revision surgeon. Cement with or without screws, modular or custom augments, and particulate or bulk graft have all been advocated for certain bone deficiencies [7, 8, 11]. For any strategy, implant stability on the host bone is vital to long-term success. A second goal of revision surgery, namely, bone stock restoration , may be accomplished through the use of bone graft. An understanding of the basic science involved in the use of allograft is exceedingly important for the revision surgeon to comprehend. Therefore, a brief review of the biology and biomechanical aspects of allograft is in order.
Basic Science of Allograft
Biology
The bone was one of the first tissue transplants performed and remains one of, if not the most, abundant tissues transplanted. Originally, autograft was used to unite fractures and fuse joints . Allograft use increased in prevalence in orthopaedic oncology as limb-salvage techniques improved. In revision hip and knee surgery, allograft bone is used when conventional methods of reconstruction are inadequate and because autograft is in short supply. As a tissue, bone has unique properties that are critical for success. Osteogenesi s is the ability to produce a new bone and is accomplished by osteoblasts. Bone proteins, such as bone morphogenic protein that are a part of the bone matrix, stimulate new bone formation through the recruitment and differentiation of pluripotent mesenchymal stem cells into osteoblasts. This characteristic is known as osteoinduction . Lastly, osteoconduction is the graft’s ability to act as scaffolding for the ingrowth of blood vessels and cells from the host bed. The process known as creeping substitution is the gradual resorption and replacement of this scaffolding with the host bone. Autograft and fresh allograft possess all of these properties. Fresh allografts, however, are rejected by the host immune system, resulting in complete graft resorption or marked delay in incorporation. Therefore, allograft used in revision joint surgery is processed and possesses the property of osteoconduction only. The success of allograft depends largely on its ability to heal to and incorporate with the host bone. Histologically, these events are similar to fracture healing. Inflammation predominates early on. Unlike autograft, in which surviving surface osteoblasts contribute bone, allograft incorporation depends on osteoblasts differentiated from pluripotent cells brought in by vasculature from the host bed. Thus, the process is similar to autograft incorporation but slower. This early phase is similar for the cancellous as well as cortical bone. Creeping substitution characterizes the incorporation of cancellous bone. That is, bone formation and resorption occur concomitantly. Eventually the entire graft may be replaced by the host bone. In the cortical bone, formation only occurs after resorption. Consequently, the graft is weaker than the normal bone for a long period of time and must be protected from excessive loading. In theory, this remodeling process eventually involves the entire structural graft. In reality, these grafts have little biologic activity outside of the graft-host junction [12].
Although animal studies have supplied most of our knowledge of the basic science of allograft, human retrievals have given the most insight into the biologic behavior of processed allografts in humans. Enneking et al. studied 16 retrieved massive human allografts that had been in situ for 4–65 months [13]. They demonstrated that union between allograft and host took place slowly at cortical-cortical junctions and more rapidly at cancellous-cancellous junctions. Internal repair was confined to the superficial surfaces and ends of the grafts and had involved only 20% of the graft by 5 years. The deep portions of the graft retained their architecture. Parks and Engh’s study of allografts in revision knee arthroplasty retrievals had similar findings with no evidence of revascularization, resorption, or remodeling beyond the graft-host union [14] (Figs. 8.3 and 8.4).



Fig. 8.3
Slab radiograph showing location and intact structure of two femoral head allografts in the proximal tibia . Note host to graft junction (arrows) (from Parks NL, Engh GA. The Ranawat Award. Histology of nine structural bone grafts used in total knee arthroplasty. Clin Orthop. 1997;345:17–23, with permission)

Fig. 8.4
Left to right: live marrow elements, live host bone, dead graft bone, avascular grafted region. The live bone is growing onto the dead graft as if it were a scaffold at the host to graft junction (Stain, hematoxylin and eosin; magnification light microscopy, ×200) (from Parks NL, Engh GA. The Ranawat Award. Histology of nine structural bone grafts used in total knee arthroplasty. Clin Orthop. 1997;345:17–23, with permission)
Ultimately, the biology depends greatly on the clinical situation and the type of graft used. As discussed, bone loss in revision surgery can be cavitary or segmental . A cavitary lesion with a well-vascularized bed is ideal for the cancellous bone, and complete incorporation is to be expected. With increasing bone loss and decreasing vascularity, a more inconsistent incorporation is to be expected. In contrast, segmental loss requiring large structural allograft relies on cortical-to-cortical contact between host and graft. The majority of the graft is surrounded by soft tissue that is usually avascular scar. Here the allograft can unite to the host bone, but there will be little if any internal remodeling of the graft [13].
Biomechanics
In reviewing the biology of allografts, we see that union with the host bone is the first step toward success. Unfortunately, failure can and does still follow all too often. Fracture of structural allograft is reported to be as high as 16.5% [15]. It goes without saying that the biomechanical behavior of the graft is of critical importance in determining success or failure. The individual factors that influence the physical properties of transplantable bone are analyzed in this section.
The ability of a graft to withstand loads is largely determined by the original properties of the bone at the time of donation. Although supply often limits surgeons’ options when choosing donor material, the factors that influence these properties should be known. For instance, bone tissue is strongest in the 20- to 39-year-old age groups and typically weakens thereafter. However, even in the 70- to 79-year-old age group, 70% to 85% of the maximum strength is maintained [16]. The surgeon can more closely control other factors, such as the method of preservation and sterilization.
The more common methods of preserving and storing specimens until they are required for implantation are freezing and freeze-drying . Both alter the immunogenicity of the graft, but freeze-drying has a more substantial effect on the physical properties [16, 17]. Freeze-drying causes little change or a slight increase in compressive strength but lowers the bending and torsional strength substantially [15, 18, 19]. Cracks have been observed in rehydrated freeze-dried specimens, which might explain the observed reduction in strength [18]. Freezing alone has little if any effect on the physical properties of the bone [18, 20]. These observations suggest that fresh frozen bone would be best when large torsional and bending loads can be expected. Clinically, this would be seen at the host-graft junction when a whole distal femur was used. Conversely, in a situation in which the graft will see primarily compressive loads, freeze-dried graft should be biomechanically sound. Most cavitary or isolated metaphyseal lesions fall into this category.
Sterilization of a graft prior to implantation can be done either of two ways. The grafts can be sterilely harvested and stored, or nonsterile grafts can be secondarily sterilized with high-dose radiation. Radiation below 3 megarads appears to cause little change in bone strength; however, above this level, significant alterations in the physical properties occur, resulting in a decrease in the compressive, bending, and torsional strength of the graft. These effects are magnified when combined with freeze-drying [19, 20].
Once retrieved, preserved, stored, sterilized, and implanted, a bone graft is subjected to load. The bone can fail under the single application of a large load if a fall or some other trauma ensues. However, fatigue failure secondary to repetitive smaller loads is more common with large allografts. Live bone is capable of remodeling when subjected to these loads. Until transplanted bone becomes vascularized, it does not have this capability. Because retrieval studies have shown that outside of the host-graft junction little remodeling occurs, it is imperative that large allografts be protected with adequate internal fixation to prevent fatigue failure. Intramedullary fixation with stemmed components is preferred over plates and screws because the stress risers made by screw holes weaken the graft, thus increasing the fracture risk.
Disease Transmission
Although extremely rare, transmission of an infectious agent through allograft bone transplantation remains a relevant concern. Most of this has centered on transmission of human immunodeficiency virus (HIV). The risk estimate for HIV transmission in 1990 was 1 in 1.6 million [21, 22]. With improved screening tools and sterilization methods and stricter donor criteria, this risk may be even less today. In the early 2000s, disease transmission from allograft bone received significant attention after a Minnesota man died from a Clostridium infection 4 days after an osteochondral transplant, and the Centers for Disease Control (CDC) uncovered 26 cases of infection from orthopaedic allograft transplants related to poor techniques in procurement, processing, and storage. Utilizing a tissue bank that is a member of the American Association of Tissue Banks reduces this risk of bacterial contamination , but these risks should be considered when counseling patients on surgical options.
Indications and Techniques
As stated before, preoperative planning combined with intraoperative findings can help the surgeon formulate the appropriate treatment strategy for dealing with bone loss. Determining the quantity, location, and extent of the bone loss is critical to a successful revision. Once the existing components have been meticulously removed using bone preservation techniques, the surgeon can evaluate whether the bony defects are contained or segmental. Contained defects are defined as defects that are surrounded by an intact cortical sleeve or rim. Uncontained defects , also known as segmental defects, are those which are not surrounded by cortical rim but rather in continuity with surrounding soft tissues.
Contained Defects
Bone deficiency in many revisions is minor and contained. After component removal, bone loss is limited to punctate cancellous defects . Minor defects have been defined differently in terms of size. In general, it is assumed that cancellous metaphyseal bone is in sufficient supply and quality to support primary implants. In these cases, defects can be filled with cement, particulate autograft from bone cuts, or particulate allograft if autograft supply is insufficient. Outcome will be similar regardless of management.
For smaller defects under 5 mm in depth that remain contained, the use of cement can be utilized to successfully fill the void [23]. This has the advantage of being readily available, given the fact that majority of revision implants are cemented. Additionally, the use of cement to fill bony contained defects can be supplemented by the use of cortical screws to enhance the strength of the construct. However, in a young patient, filling the bony defect with morselized allograft can restore bone stock facilitating any future revision that might take place.
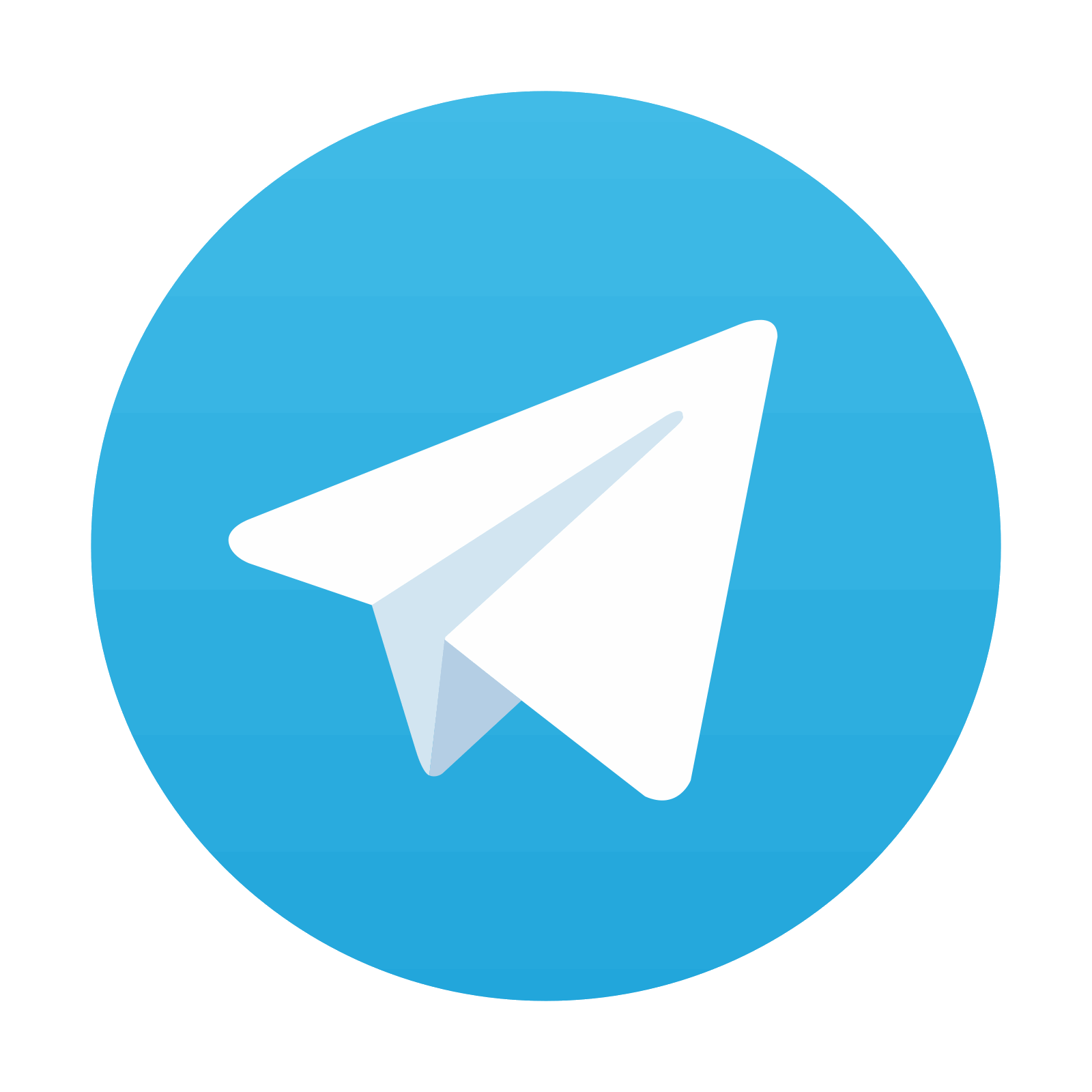
Stay updated, free articles. Join our Telegram channel
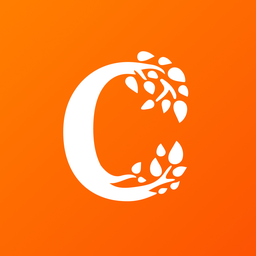
Full access? Get Clinical Tree
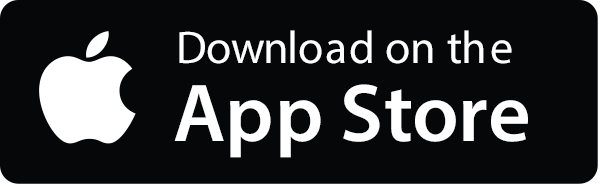
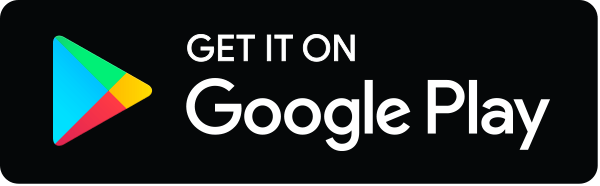