The Osteochondral Unit and Biology of Cartilage Repair
The function of diarthrodial joints relies on the health and integrity of the osteochondral unit, which encompasses the composite tissue of articular cartilage, calcified cartilage layer and subchondral bone. , Alterations in any of these tissue components can lead to disruption of normal joint homoeostasis and function and contribute to various pathological conditions and afflictions.
Articular Cartilage
Normal articular cartilage contains specialised hyaline cartilage of diarthrodial joints and functions by facilitating the transmission of loads and supporting very low levels of friction between the articulating surfaces under physiological conditions. , Articular cartilage represents a smooth surface of approximately 1 to 4 mm in thickness that overlies the subchondral bone and contacts synovial fluid. , The articular cartilage itself is devoid of vasculature, nerves and lymphatics and contains chondrocytes, specialised cells that respond to various mechanical and chemical signals to maintain matrix integrity. Cartilage contains a solid component composed of extracellular matrix and proteins that supply the structural framework and a fluid component composed of water, solutes, metabolites, small proteins and a high concentration of cations (Ca 2+ , K + , Na + , CL – ). The solid and fluid components contribute to the lubrication, mechanical stiffness, and load distribution functions of the articular cartilage. , Loading of the joint leads pressurises the interstitial fluid, which plays a major role in supporting compressive loads and preventing deformation of the solid matrix component. Because of the high specialisation of the tissue, limited mitotic ability of the chondrocytes and lack of vascularity, articular cartilage lacks the intrinsic repair potential that other tissue types such as bone or skin maintain through adulthood.
Components of Articular Cartilage
The articular cartilage tissue component is composed of the superficial, middle transitional, deep and calcified zones ( Fig. 21.1 ). Each zone contains of a cellular and extracellular matrix component primarily composed of proteoglycans (5% to 10%), collagen (10% to 20%) and water (68% to 85%). Proteoglycans are macromolecules composed of a protein core and polysaccharide chains called glycosaminoglycans . Aggrecan is the primary proteoglycan in articular cartilage that contributes to its net negative charge and maintains the high osmotic pressure, which is responsible for its mechanical function via fluid pressurisation. The matrix is primarily composed of collagen types II (90%), VI (1% to 2%), IX (1% to 2%) and XI (2% to 3%), which contribute to the matrix tensile strength and stiffness. The unique matrix architecture and composition vary with depth of the articular cartilage, with the respective chondrocyte density and morphology predicating its function and mechanical properties. ,

The zonal cartilage organisation can also be defined by regions based on their distance from the chondrocytes – the pericellular, territorial and interterritorial regions. , , The region most closely surrounding the chondrocytes is the pericellular matrix, which is composed of noncollagenous proteins and characterised by its high proteoglycan content. The territorial region consists of thin collagen fibrils that surround the individual regions of pericellular matrix and chondrocytes or clusters of multiple chondrocytes and their pericellular matrix. , The third, interterritorial region is the primary region of the articular cartilage and contains larger collagen fibrils whose alignment varies with depth and zone of the cartilage. ,
Zone Organisation
The zone variations ( Fig. 21.1B ) are defined by differences in composition, collagen alignment and cross-linking and chondrocyte size, shape, function and metabolic activity. , The superficial zone is defined as the outermost layer of the articular cartilage contacting the synovial cavity and fluid, composed of the outer 10% to 20% of the articular cartilage total thickness. , This layer consists of densely packed flattened chondrocytes and collagen fibres oriented parallel to the articular surface, which contributes to the tensile strength of the articular cartilage. , , , The superficial cells are responsible for secreting proteoglycan 4 (PRG4), a glycoprotein that supports lubrication. , This zone is further characterised by high fibronectin, water and elastin content. , The middle transitional zone (approximately 40% to 60% of total articular cartilage thickness) contains thicker collagen fibres and chondrocytes with a spherical morphology randomly distributed throughout the matrix. , , , , The middle zone is characterised by high aggrecan and collagen type II content, which contributes to its increased compressive modulus. , The collagen fibre orientation in the middle transitional zone presents an obliquely arranged alignment known as part of the arcades of Benninghoff. , , The deep zone, approximately 30% to 40% of the total articular cartilage thickness above the tidemark of the calcified cartilage region, contains fibres arranged perpendicular towards the articular surface with rounded chondrocytes arranged in clusters or columns. , , , This zone contains the lowest cell density and high proteoglycan content contributing to the high compressive modulus of this region. , The calcified cartilage zone is a thin layer with thickness of approximately 50 m to 250 μm, varying by individual, location and mechanical loading. This biocomposite dense mineralized tissue and extracellular matrix (collagen type II, glycosaminoglycan, collagen type X) is separated from the articular cartilage by a histologically defined tidemark and functions to attach the noncalcified articular cartilage to the subchondral bone. , , This region contains a gradient of mineral content with an exponential increase of this mineral content from the adjacent articular cartilage to the subchondral bone, conveying the mechanical properties of this region. , ,
In addition to variations in collagen alignment, the fluid and solid components of articular cartilage vary between zones, imparting their particular mechanical properties ( Fig. 21.1C ). Water content is most at the surface at approximately 80% wet weight (ww) and decreases linearly towards the subchondral bone to approximately 40% to 60% ww. The collagen content is highest in the superficial layer 86% dry weight (dw) and decreases with depth to approximately 67% dw in deep zone. Proteoglycans, the main noncollagen protein, are also depth dependent, with the lowest content at the superficial zone approximately 15% dw and increased content in the middle approximately 25% dw and deep zones 20% dw. , , , In contrast, the porosity of the cartilage, or interstitial fluid content, is highest in the superficial zone and lowest in the middle and deep zones. Compared with the superficial zone with its high collagen content and increased tensile strength, the middle and deep zone contain increased proteoglycan and water content, with greater compressive strength. ,
Chondrocytes
Chondrocytes, similar to osteocytes and other cell types, have the ability to respond to mechanical or physical stimuli. The ability of cells to convert mechanical forces into biochemical signals occurs through mechanotransduction, where chondrocytes respond to various physical stimuli through biosynthesis and matrix remodelling. Depending on their zone, chondrocyte subpopulations in the superficial and deep regions vary in cell density and morphology, as well as their biosynthesis, metabolic activity, cytokine activity and gene and protein expression, which is closely associated with matrix constitutions. , , , , Amanatullah et al. demonstrated a dramatic difference in the expression of extracellular matrix genes between superficial and middle zones. PRG4, a lubricant protein also identified as lubricin or superficial zone protein (SZP), is primarily expressed and secreted by the superficial zone chondrocytes. , , Collagen type II expression and glycosaminoglycan production are increased in the middle and deep zones, whereas PRG4 secretion is decreased compared with the superficial zone. , , , ,
Synovial Fluid
Synovial fluid contributes to the extremely low friction and wear properties of the articular cartilage surface through fluid film lubrication and boundary lubrication mechanisms. Synovial fluid is produced by the synovium and is composed of water, inorganic salts and macromolecules, hyaluronic acid, lubricin and aggrecans, which contribute to the boundary lubrication. Hyaluronic acid (HA) is a high molecular weight polysaccharide that contributes to the high viscosity of the synovial fluid and had been recognised as the main boundary lubricant. It was later shown that enzymatic removal of hyaluronic acid did not affect the lubricant properties. , Boundary lubricant properties were found to be influenced by lubricin, a glycoprotein identical to SZP or PRG4 that is present in both the synovial fluid and articular cartilage surface. , More recently, the synergistic interaction of boundary lubricants HA, phospholipids and lubricin has been shown to play a major role in boundary lubrication of a healthy synovial joint.
Subchondral Bone Architecture and Function
Subchondral bone plays a critical role in the healing of osteochondral defects and also may contribute to the development and progression of chondral diseases. Subchondral bone, composed of mineral, collagen and water, varies substantially in thickness, architecture and composition between individuals and anatomical locations. The subchondral bone consists of a subchondral bone plate, a thin layer of cortical-like bone with haversian structures, between the calcified cartilage zone and underlying trabecular bone. , The subchondral bone plate, once thought to an impermeable barrier, is now recognised to be permeable to small molecules and contribute to the nutrition of articular cartilage. , , , The vasculature of the subchondral bone is supplied through vessels running through mature osteons. Channels containing irregular sinusoids and veins have been identified through the subchondral bone region. Extensions of these channels and vessels occasionally penetrate above the subchondral bone and into the calcified and uncalcified cartilage, facilitating perfusion to this region. , , , The vascularisation of the subchondral bone varies individually and is influenced by load bearing. Subchondral bone architecture and remodelling is further influenced by load and follows Wolff’s law by responding and adapting to changes in joint load. , , The adaptations of the subchondral bone are mediated through highly regulated cellular processes of bone remodelling, which involves the coordinated activity of osteoblasts, bone cells that contribute to bone formation by secreting collagen type I, and osteoclasts, bone cells involved in resorption by removal of mineralized extracellular matrix. , Osteocytes are embedded in the bone matrix and play a major role in regulating the bone remodelling process by responding to mechanical and soluble mediators.
Physiological Transport
Despite the avascular properties of the cartilage, transport of (macro-)molecules, nutrients and oxygen to the chondrocytes is supported through diffusion and convention of the interstitial fluid through the extracellular pore spaces of the cartilage. , The rate of diffusion is influenced by the structure and composition of the extracellular matrix in the various cartilage zones. The synovial fluid was traditionally thought to be the main contributor of nutrients and metabolites to the articular cartilage. With a better understanding of transport mechanisms between the subchondral bone and articular cartilage, it is now understood that the vasculature of the subchondral bone contributes substantially to the nutrient requirements of the articular cartilage. Vascular channels between the interface of the subchondral bone and calcified cartilage provide a mechanism of communication and molecular diffusion between the two compartments. The close proximity of the uncalcified articular cartilage and subchondral bone layers via vascular microchannels and the irregular anatomy of the bone–cartilage interface supports the communication and diffusion of nutrients, oxygen and other small molecules between the subchondral bone and articular cartilage. Several studies have identified prolongations of the uncalcified cartilage dipping through the calcified region and interfacing with the subchondral bone and marrow spaces. , , The irregular structure of the interface may facilitate a mechanism of molecular communication between the uncalcified articular cartilage and subchondral bone layers. , The calcified cartilage layer is semipermeable and provides transport of small molecules from the subchondral bone to the articular cartilage. Several studies have demonstrated small molecules can diffuse between the marrow space and articular cartilage. , , Maintenance of normal physiological transport to subchondral bone via surrounding host bone is important to the maintenance of articular cartilage.
Biology of Osteochondral Repair
Superficial and full-thickness chondral defects, defects that extend through superficial or all zone of the articular cartilage, lack the ability to spontaneously repair and heal. , The stages of classic wound healing (necrosis, inflammation, proliferation, remodelling) and the orchestra of reparative bioactive molecules and cells do not occur in the injured chondral tissue as a result of the lack of vasculature. , , The repair process of chondral defects is dependent on the surrounding chondrocytes, which have limited mitotic and migratory ability. , The native matrix components of the articular cartilage impede the migration of chondrocytes from adjacent healthy tissue and limit the diffusion of matrix proteins. , , Cell death adjacent to the defect also creates a metabolically inactive zone and limits the ability of surrounding chondrocytes to migrate and contribute to repair. , However, osteochondral defects that extend through the articular cartilage and the subchondral plate do stimulate a more classical repair response ( Fig. 21.2 ). , The repair response of the osteochondral and chondral lesions will vary by various factors, including age, species, defect size, depth and location. , , In general, defects penetrating the subchondral bone initiate a fibrous clot comparable to the fracture repair process. , The fibrin clot contains platelets that release growth factor and cytokines that stimulate vascular invasion, cell migration, proliferation and differentiation. During the initial stages of response, the adjacent articular cartilage tissues demonstrate signs of degeneration, proteoglycan loss and chondrocyte clustering. Recruitment and migration of reparative mesenchymal cells and invasion of capillary vessels originating from the adjacent marrow spaces occurs within the first week. , , , The fibrin clot dissolves and a fibrous structure serves as scaffold for the undifferentiated cells. Studies have reported that reparative cells may also originate from other tissues, including the synovium, peripheral blood and articular cartilage. , In the upper portion of the defect, the neocartilage or hyaline-like chondroid tissue, containing substantial amounts of collagen type II, proteoglycans and collagen type I, is formed adjacent to the damaged cartilage within the initial weeks of repair. , , , The formation of the chondroid tissue has been attributed to undifferentiated progenitor cells that migrate from the bone marrow and activation of cartilage progenitor cells from damaged cartilage. , Woven bone, containing unorganised randomly oriented collagen fibrils, is synthesised by osteoblasts in the lower region of the defect in adjacent marrow spaces of trabecular bone. , , Chondroid tissue extending to the surface is formed within the initial weeks after injury. This repair tissue contains substantial amounts of collagen type II and chondrocyte-like cells with a rounded appearance. Despite the formation of hyaline-like tissue, the repair tissue fails to form the highly organised zonal structure and composition of normal articular cartilage. During this time frame, endochondral bone formation is observed in the lower portion of the defect and osteoblasts continue to synthesise woven bone in adjacent marrow. , , The endochondral ossification consists of chondrocyte differentiation, hypertrophy, degeneration and subsequent bone formation in the deep regions of the defect.
In the later stages of repair, the chondroidlike tissue is remodelled with fibrocartilage through increased synthesis of collagen type I and a loss of chondrocyte-like cells. , , , , Degenerative changes of the repair tissue are observed between 10 and 24 weeks, including diminution of proteoglycan, fissuring near the surface and hypocellularity. , In the deeper regions, extensive bone formation is observed and the appearance of a tidemark occurs. , By 6 to 12 months, the tissue is mostly fibrous and demonstrates continuous degradation with surface fibrillation and acellular areas. , , , , , Fibrocartilage does not provide the mechanical properties of articular cartilage and is incapable of withstanding the biomechanical environment of a loaded joint long term. , The higher coefficient of friction of fibrocartilage further impedes motion and accelerates degeneration. This is reflected by the continuous fibrillation and degeneration that occurs after fibrocartilage formation, typically within a year. , , In the later stages of repair, the subchondral region reaches a normal level and is organised with formation of a compact bone plate, osteon development and lamellar bone or bone with organised collagen fibrils that are clustered in parallel arrays. , , , A number of animal models investigating spontaneous repair observed subchondral bone plate migration and overgrowth in the later stages, which may be attributed to both endochondral ossification and intramembranous bone formation. , , The timeline of repair, overall bone healing process of the subchondral bone and observation of subchondral plate migration is greatly influenced by the animal species and defect size. The clinical impact of subchondral plate upward migration and alterations of subchondral architecture on repair and outcomes is unclear but ostensibly can be responsible for intralesional osteophyte formation.
In a number of studies investigating the natural repair process, the repaired tissue fails to integrate with the native articular cartilage. , , , , This may be attributed to the limited remodelling of the residual cartilage and cell death and deformation of the residual and adjacent surrounding articular cartilage. , , , The lack of integration may contribute to the poor long-term quality of a fibrocartilaginous repair. Strategies using proteolytic enzymes may augment the removal of the necrotic residual tissue and provide a mechanism for surrounding chondrocytes entrapped in the matrix to contribute to repair.
Molecular Factors That Contribute to Repair
After injury, various molecular signals have been shown to be involved in response to the injury including members of the insulin-like growth factor family (IGF), fibroblast growth factor family (FGF), transforming growth factor-β family (TGF-β) and platelet-derived growth factor (PDGF). , , , , A number of growth factors have been shown to contribute to the intrinsic repair response of cartilage. , Bos et al. measured the spatial and temporal expression of growth factors in a rabbit cartilage wound model. High levels of TGF-β were observed 3 days after injury, whereas other growth factors, IGF-1 and FGF-2, known to influence chondrocyte function and metabolism, showed peak expression at day 7. , The role of various growth factors and cytokines in the cartilage repair process have been investigated in a number of studies. , During the early stages of osteochondral repair, the fibrin clot containing platelets contributes to the releases of vasoactive mediators and growth factors, including TGF-β and PDGF. TGF-β has been reported to influence cartilage proteoglycan and collagen synthesis. Outcomes of blocking TGF-β receptors in arthritic animal model showed a significant reduction in proteoglycan content. Furthermore, administering TGF-β in an inflamed knee increased proteoglycan content, which may contribute to the enhancement of repair. Another member of the TGF-β superfamily, bone morphogenetic protein-2 (BMP-2), has been shown to stimulate matrix synthesis, increase cartilage matrix and reverse chondrocyte dedifferentiation turnover. , FGF-2 of the FGF family promotes anabolic pathways and reduces catabolic enzyme activity. FGF-2 has been shown to also have a chondroprotective effect.
Pathology and Repair
Osteochondral tissue has an innate microstructure that has ramifications not only for its function but also in turn for its healing in response to injury, which has implications for surgical restorative options. It is generally accepted that partial-thickness articular cartilage lesions do not spontaneously heal in the skeletally mature individual and that full-thickness lesions heal with mixed repair tissue that is inferior to native articular cartilage in function and durability. Determining and quantifying the depth of the (osteo-)chondral lesion thus become paramount for devising and implementing successful treatment strategies. To this end, several classification systems have been suggested, of which the International Cartilage Repair Society (ICRS) classification system is one of the most comprehensive and commonly accepted ( Fig. 21.3 ). Although the superficial layer of articular cartilage with its lamina splendens likely contributes significantly to the maintenance of boundary lubrication via production of PRG4, fibrillation of that zone (ICRS grade 1) is not routinely or reliably restored but usually treated with benign neglect or simple debridement when encountered intraoperatively. Isolated partial, higher grade articular cartilage lesions can present a treatment dilemma. The microstructure of the cartilage’s collagen matrix is considered essential to conveying its viscoelastic properties by providing a relatively stiff lattice for hydrated proteoglycan side chains to be pressurised against. Although the loss of structural integrity ostensibly leads to increased water content and swelling of the extracellular matrix, followed by egress of proteoglycans and propagated fibrillation, it is important to remember that, overall, the natural history of articular cartilage lesions remains unpredictable and that articular cartilage is aneural and thus unlikely to be a primary pain generator. Thus, there is no role for preventative surgery to address clinically quiescent cartilage lesions that are commonly, and usually incidentally, encountered during routine arthroscopy. However, partial-thickness chondral lesions may contribute to overall symptomology focally via mechanical effects on the adjacent subchondral bone or on the synovial environment. Bone marrow lesions (BMLs) on magnetic resonance imaging MRI that correlate with chondral lesions can give important clues to the clinical significance of both partial- and full-thickness chondral injuries alike. In the absence of frank osteochondral trauma, different causes for BMLs have been proposed, implicating either repair phenomena or increases in intraosseous pressure that may occur secondary to increased pressure transmission or inadequate subsurface support. Articular cartilage relies on an intact subchondral plate for containment and to maintain adequate intrachondral hydrostatic pressure. Injuries to the osteochondral unit can be associated with microfractures of the subchondral plate and bone, leading to decreased containment and forced egress of interstitial fluid from the cartilage into the subchondral bone, with directional fluid pressure that is inversely related to porosity. High local fluid pressure can lead to osteolysis and excitation of pain fibres and sclerosis of surrounding bone, further increasing strain on overlying cartilage in a vicious cycle. High-grade (ICRS grades 3 or 4) cartilage lesions can expose innervated subchondral bone to direct loads during weightbearing or from increased synovial fluid pressure, although this effect can be mitigated if the lesion is contained, meaning shouldered by healthy cartilage tissue that provides relative stress shielding and prevents direct pressure being exerted on the exposed subchondral bone ( Fig. 21.4 ). When considering the size of a chondral lesion, shouldering and the relative, probably more so than overall, size of a lesion are important aspects in choosing appropriate treatment options.
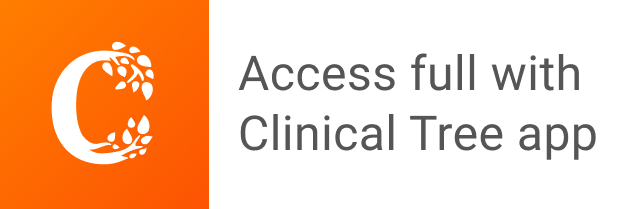