4.1 Polytrauma: pathophysiology, priorities, and management
To access the videos, please follow the URL link
1 Definition
Polytrauma has been defined as “a syndrome of multiple injuries exceeding an Injury Severity Score (ISS) of 16 with sequential systemic reactions that may lead to dysfunction or failure of remote organs and vital systems that have not been directly injured”. However, an international consensus in 2014 [1] suggested that polytrauma be redefined as the presence of two injuries that are greater or equal to 3 on the Abbreviated Injury Scale (AIS) and one or more of the following additional conditions:
Hypotension (systolic blood pressure ≤ 90 mm Hg)
Unconsciousness (Glasgow coma scale [GCS] score ≤ 8)
Acidosis (base deficit ≤ 6.0)
Coagulopathy (partial thromboplastin time ≥ 50 seconds or International Normalized Ratio ≥ 1.4)
Age (70 ≥ years)
2 Importance of fractures
At the beginning of the 20th century surgical stabilization of long-bone fractures in multiple-injured patients was not routinely performed. The major reason behind this approach was the fear of fat embolism syndrome (release of fat and intramedullary [IM] contents into the peripheral circulation). Nowadays, the consequences of this syndrome are believed to be secondary to the breakdown of fat to free fatty acids, the release of toxic mediators, and subsequent immunoinflammatory reactions leading to an increased vascular permeability. This results in alveolar hemorrhage, edema, extravasation of polymorphonuclear leukocytes, and the development of respiratory insufficiency (adult respiratory distress syndrome [ARDS]) [2].
The first evidence for the beneficial effects of long-bone fracture stabilization became available from World War I with use of the Thomas splint for the management of femoral shaft fractures resulting in improved survival rates. Subsequently, the advent of antibiotics together with advances in intensive care and anesthesia, implant design, and the implementation of standardized fracture fixation techniques by the AO supported a more operative approach for fracture fixation. However, for many decades, the philosophy prevailed that the injured patient was “too sick to operate on” and patients were treated with skeletal traction and enforced bed rest. However, in the early 1980s a prospective randomized study by Bone et al [3] demonstrated the benefits of early femoral fracture fixation, with a reduction in the incidence of respiratory failure leading to a reduced length of stay in intensive care and in hospital. This study set the foundation for the philosophy of early total care (ETC) that subsequently prevailed. Consequently, the previously held belief among surgeons that the patient was too sick to operate on was now replaced with the opposite view that the patient was “too sick not to operate on”. Early total care became the optimum treatment in surgery for fracture fixation and further developments in intensive care medicine reinforced early fracture fixation in patients with polytrauma.
The ETC strategy was widely used but several reports in the literature described adverse outcome after ETC in some groups of patients with an increased incidence of ARDS and multiple organ failure. In response to these observations, the concept of damage-control orthopedics (DCO) for the management of multiple-injured patients was developed. This approach was based on the principle of “damage limitation” [4].
Early total care and DCO became a hot topic of discussion between European and North American surgeons as to which multiple-injured patient would benefit most from either surgical strategy.
Recently, the concept of early appropriate care was introduced to emphasize that fracture fixation should not take place during resuscitation but should be delayed a short time until the patient is fully resuscitated with a return to normal physiological parameters. Early appropriate care also considers the surgical fixation of unstable pelvic and spinal fractures during this phase. Thus, it is now recognized that ETC and DCO are complementary to each other and used for different groups of patients [5].
Although ETC and DCO made a major impact on trauma care, it took several years to appreciate the fact that unstructured management of trauma patients is associated with avoidable death and disability. The introduction of trauma centers in the US and the more recent development of regional trauma networks (RTN) in Australia and England have demonstrated the beneficial effect of organized trauma care systems on outcomes. The structure of the national system in England is based on the fundamental components suggested by the Committee on Trauma of the American College of Surgeons consisting of:
Leadership (at all levels of trauma care delivery)
Prehospital and in-hospital triage
Designated and accredited trauma care facilities (major trauma centers (level I), trauma units (level II), local emergency hospitals (level III), transport services, rehabilitation units)
Human resources (planning and development, administrative and clinical teamwork)
Education-prevention-public awareness, good communication (at all levels of the trauma system)
Rehabilitation plus data collection
Audit and research with quality assurance monitoring [6]
Fractures have an important impact on the severity of the systemic reaction to trauma due to:
Hemorrhage: prolonged states of shock as well as exsanguinating hemorrhage are frequently associated with unstable pelvic ring injuries, femoral shaft fractures, multiple long-bone fractures, and open injuries.
Contamination: open fractures must always be considered as contaminated. If a wound can only be debrided after some delay or if debridement is not radical enough, the wound will become a perfect culture medium for bacteria resulting in local and sometimes systemic infection.
Dead, ischemic tissue with a hypoxic zone at the margin: in unstable, displaced open fractures, especially after high-energy impact, a radical soft-tissue debridement is necessary as soon as possible to remove this source of proinflammatory mediators.
Ischemia-reperfusion injury: prolonged hypovolemic shock and compartment syndrome related to fractures with or without vascular injuries are prone to ischemia-reperfusion injury with microvascular damage due to oxygen radicals. Blunt tissue contusions may activate xanthine oxidase; ischemia will produce the substrate xanthine/hypoxanthine, and reperfusion will add the co-substrate oxygen resulting in the formation of destructive free-oxygen radicals. A dangerous triad is established.
Stress and pain: unstable fractures cause pain and stress which, via afferent input to the central nervous system, stimulate a neuroendocrine, neuroimmunological, and metabolic reflex arcs [7].
Interference with intensive care: unstable fractures prevent effective patient postures (eg, upright chest), and pain-free patient movement.
In general terms the major aims of fracture management in the polytrauma patient are:
Control of hemorrhage
Control contamination sources
Prevention of ischemia-reperfusion injury
Pain relief
Facilitate ventilation, nursing, and physiotherapy
These aims can be fulfilled by hemostasis, debridement, fasciotomy or revascularization, fracture fixation, and tension-free wound coverage.
For stabilization of long bones, external or internal fixation are options depending on the circumstances.
3 Pathophysiology
Trauma initiates a series of physiological reactions involving the cardiovascular, immune, and coagulation systems with the aim to maintain homeostasis and survival. The cardiovascular system initially exhibits a hypodynamic flow secondary to hypovolemic shock, which becomes hyperdynamic when resuscitation has been successfully completed [8]. Activation of the immune inflammatory system results in the development of both the systemic inflammatory response syndrome (SIRS) and the counter antiinflammatory response syndrome (CARS). Under ideal circumstances a fine balance between those two immune reactions is maintained and the recovery is uneventful. An exaggerated SIRS might lead to ARDS, multiple organ dysfunction syndrome (MODS), or even death while a decompensated CARS could contribute to immunosuppression and development of early sepsis [9]. The response of the coagulation system to trauma and hemorrhage is complex and involves a controlled interaction between the vasculature, circulating platelets, coagulation proteins, and the fibrinolytic mechanism. Coagulation is initiated either by reactions between components of the blood alone (the intrinsic pathway) or by reactions that also involve tissue components (the extrinsic pathway). Normally, a fine balance is sustained between keeping blood in a fluid state and the development of clots.
3.1 Primary response to severe trauma
Local and systemic reactions are initiated in the immediate aftermath of severe trauma. Fractures, soft-tissue injury, organ damage (lung, liver, bowel, etc), hypoxia, acidosis, and painful stimuli all contribute to these reactions. The main physiological response aims at stopping hemorrhage and maintaining blood flow to vital organs.
The first response to injury is characterized by the release of adrenocorticosteroids and catecholamines by the neuroendocrine system inducing an increase in heart rate and respiration rate together with leukocytosis and fever. Stimuli from aortic and carotid receptors trigger the renin-angiotensin system in an effort to maintain blood pressure through vasoconstriction. At the same time the metabolic rate is reduced to minimize energy expenditure [10].
The first clinical priority is to stop hemorrhage, prevent hypoxia and hypercarbia (which result in acidosis), and avoid hypothermia. All of these result in secondary damage to key organs, such as the brain and they are considered precursors of SIRS.
Following trauma, numerous adaptations in inflammatory and immunological functions occur. Proinflammatory and antiinflammatory mediators are released regulating cellular and vascular responses. Polymorphonuclear (PMN) granulocytes, monocytes, and lymphocytes are activated and elicit local molecular responses associated with vascular endothelial adhesion. This adhesion process is mediated by the expression of adhesion molecules and is vital for the subsequent extravasation of PMN leukocytes. If this occurs systemically, rather than at the local site of injury, PMN leukocytes lose their autoregulation and release toxic enzymes causing remote organ injury in the form of ARDS or MODS. Failure to rapidly restore normal physiological parameters can lead to dysregulation of the immune system, paving the way for an exaggerated systemic inflammatory response and, at a later stage, immune paralysis. Thus, many of the serious, early complications of polytrauma, such as ARDS, MODS, sepsis, and thromboembolism are now considered to be associated with immune dysfunction. Interleukin-6 (IL-6) is believed to be a useful marker for assessing these alternations of the immune system, as it has been found to have a consistent pattern of expression and plasma half-life. A cutoff value of 200 pg/dL was shown to be significantly diagnostic of an “SIRS state”. Significant correlations between adverse events and both the IL-6 level and SIRS have been observed [11].
Recently, the ongoing quest to identify biomarkers of the immune responses following trauma has led to the recognition of a large family of mediators, the so-called damage-associated molecular patterns (DAMPs). Alarmins and pathogen-associated molecular patterns (PAMPs) are part of DAMPs and represent danger signals capable of activating innate immune responses after trauma. Their pathophysiological contribution in trauma-related induced systemic activation is currently under further investigation and is not yet fully understood [12].
3.2 Coagulopathy of trauma
Coagulopathy is common after severe trauma
*Several names have been used to describe this coagulation disorder as acute traumatic coagulopathy, early coagulopathy of trauma, acute coagulopathy of trauma-shock, trauma-induced coagulopathy and trauma-associated coagulopathy.
and develops early, often before the patient arrives in hospital.It is due to a combination of factors including:
Hypovolemic shock due to bleeding
Damage to vascular endothelium
Thrombin-thrombomodulin complex generation within injured tissues
Decreased activated protein C
Activation of anticoagulant and fibrinolytic pathways
Hypothermia
Additionally, hemodilution resulting from resuscitation with high volume of crystalloids can also lead to defective coagulation [13].
Coagulopathy along with the presence of acidosis and hypothermia is termed the “lethal triad” and is associated with an increased mortality rate ( Fig 4.1-1 ). Early recognition and treatment of trauma-induced coagulopathy is essential to reach successfully the end points of resuscitation.

3.3 Response to surgery: “the second hit”
The primary response to trauma involves the upregulation of several immune-physiological processes to maintain homeostasis and survival. The release of mediators and the induction of SIRS is dependent primarily on the severity of the trauma sustained (the “first-hit phenomena”). Any subsequent activation of various molecular cascades during therapeutic or diagnostic interventions, surgical procedures, and posttraumatic or postoperative complications are termed “second” or “third” hits ( Fig 4.1-2 ) [14].

It has been clearly shown that early, prolonged surgical interventions (second hits) are associated with increased risk of bleeding and stressful stimuli capable of magnifying the already evolving SIRS. In some cases, this may become uncontrollable and lead to the development of ARDS and MODS. While we cannot influence the response to the first hit, the surgeon with organized resuscitation and careful timing and planning of surgical interventions can reduce the endogenous physiological responses to this second hit, thus minimizing the risk of complications [15]. The application of the concept of damage-control surgery (DCS) in this setting is useful and life saving. Both conventional and immunological markers can quantify the presence of SIRS on admission. Table 4.1-1 includes the conventional parameters defining SIRS. Alternately, on days 0 and 1 after major trauma, levels of IL-6 > 200 pg/dL are associated with a SIRS state [11].
Body temperature | > 38 or < 36° C |
Heart rate | > 90 beats/min |
Respiratory rate | > 20/min or PaC02 < 32 mm Hg |
White blood cell count | > 12,000 or < 4000 cells/mm3 |
4 Resuscitation
Hemorrhage is the most common cause of preventable death following trauma. Resuscitation protocols emphasize that early control of hemorrhage, including immediate control of external hemorrhage—cABC is key to patient survival.
It is paramount that the physician clinically assesses the extent of traumatic hemorrhage using a combination of patient physiology, anatomical injury pattern, mechanism of injury, and the patient′s response to initial resuscitation. It has been estimated more than 50% of polytrauma patients are transfused with more than 15% of them receiving substantial transfusions [16].
A patient in hemorrhagic shock with an unidentified source of bleeding should undergo immediate assessment of the chest, abdominal cavity, and pelvic ring, which represent the major sources of occult, acute blood loss in trauma.
Currently, in organized trauma care systems, computed tomographic (CT) scanners have replaced conventional radiographic imaging techniques (plain x-rays and ultrasonography) during the primary survey. Early whole-body (trauma) CT (WBCT) allows identification of the source of hemorrhage and targeted surgery or interventional radiology to stop the bleeding. The number to treat is just 17: for every 17 patients with an ISS >16 undergoing trauma CT, there will be one additional survivor and the sicker the patients, the more they are likely to benefit from early CT scan [17].
However, it is important to note that there must be a clear clinical indication for WBCT as it involves a significant dose of radiation. The WBCT should not be used as a “screening tool” based on mechanism of injury alone.
Serum lactate or base deficit measurements are sensitive tests to estimate and monitor the extent of bleeding and shock. The amount of venous lactate produced by anaerobic glycolysis is an indirect marker of oxygen debt and tissue hypoperfusion; the severity of arterial blood base deficit values offer an indirect estimation of global tissue acidosis due to impaired perfusion. Standard coagulation monitoring comprises the early and repeated determination of PT, APTT, platelet counts, and fibrinogen.
Detection of coagulation abnormalities with viscoelastic testing has recently been introduced. Early variables of clot firmness assessed by viscoelastic testing have been reported to be good predictors for the requirement for massive transfusion and for mortality in trauma patients [18]. Portable coagulometers and thromboelastometry allow “point of care” testing in the trauma room, critical care, or operating room and provide real-time data on coagulopathy to guide patient management.
Systolic blood pressure of 80–90 mm Hg should be the target until major bleeding has been stopped in the initial phase following trauma without brain injury.
In the presence of a traumatic brain injury (GCS ≤ 8), maintenance of mean arterial pressure ≥ 90 mm Hg is recommended. This is best achieved by intravenous administration of small (250 mL in an adult) boluses of warmed crystalloid and avoiding large volumes of these fluids if at all possible, as this is associated with dilutional coagulopathy and MODS.
It must be emphasized that this approach of “permissive hypotension” must be time-limited. Rapid hemorrhage control is essential.
Early application of measures to reduce heat loss and keep the hypothermic patient warm are essential to restore and maintain normothermia.
In patients with ongoing bleeding, massive transfusion protocols have been developed to allow prompt administration of red blood cells, fresh frozen plasma and platelets. The aim is to replace blood loss and coagulation factors that are being consumed. Latest evidence suggests that the optimal ratio of blood, FFP, and platelets is 1:1:1 and this is associated with improved survival rates [19]. Thromboelastography and fibrinogen levels together with standard coagulation tests may be used to guide the administration of additional fibrinogen concentrate or cryoprecipitate. The hematologist has become an integral member of the trauma team.
Massive transfusion is defined as the replacement of a patient′s total blood volume within 24 hours, or as the acute administration of more than half the patient′s estimated blood volume per hour.
The goal of treatment is to promptly restore the blood volume and to preserve the composition of blood with regard to clotting and oxygen-carrying capacity, biochemistry, and oncotic pressure. At the start of resuscitation, cross-match, coagulation tests, full blood count, and biochemistry must be performed. Hospitals receiving patients with major trauma should have a massive transfusion protocol in place. These will vary according to local resources but should allow immediate transfusion of group O-negative blood with rapid availability of blood (packed red blood cells), thawed fresh frozen plasma and platelets in a 1:1:1 ratio. All transfusions should be warmed.
Pharmacological agents have also been used as adjuncts to hemorrhage control. The CRASH-2 trial demonstrated that tranexamic acid, an antifibrinolytic drug with a good safety profile, administered within 3 hours of either blunt or pen etrating trauma, significantly reduces transfusion requirements and mortality. Early administration is essential and ideally it should be given prehospital or early after arrival at hospital. A loading dose of 1 g of tranexamic acid is administered, followed by an infusion of 1 g over 8 hours [20].
Lately, the concept of damage-control resuscitation (DCR) has been introduced in the civilian trauma setting. The origin of DCR comes from the military′s experience of management of major hemorrhage during recent conflicts in Afghanistan and Iraq. Damage-control resuscitation was defined as “a systematic approach to major trauma combining immediate control of external hemorrhage with a series of clinical techniques from point of wounding to definitive treatment to minimize blood loss, maximize tissue oxygenation, and optimize outcome” [21]. The main components of DCR are external hemorrhage control, time-limited permissive hypotension, limitation of crystalloid administration with early use of blood and blood products (massive transfusion protocols), early use of tranexamic acid, preventing hypothermia, and early DCS.
A multidisciplinary approach to the management of the multiple-injured patient remains the basis of ideal patient care.
Development and implementation of evidence-based management protocols reduces variation and improves both process and outcome.
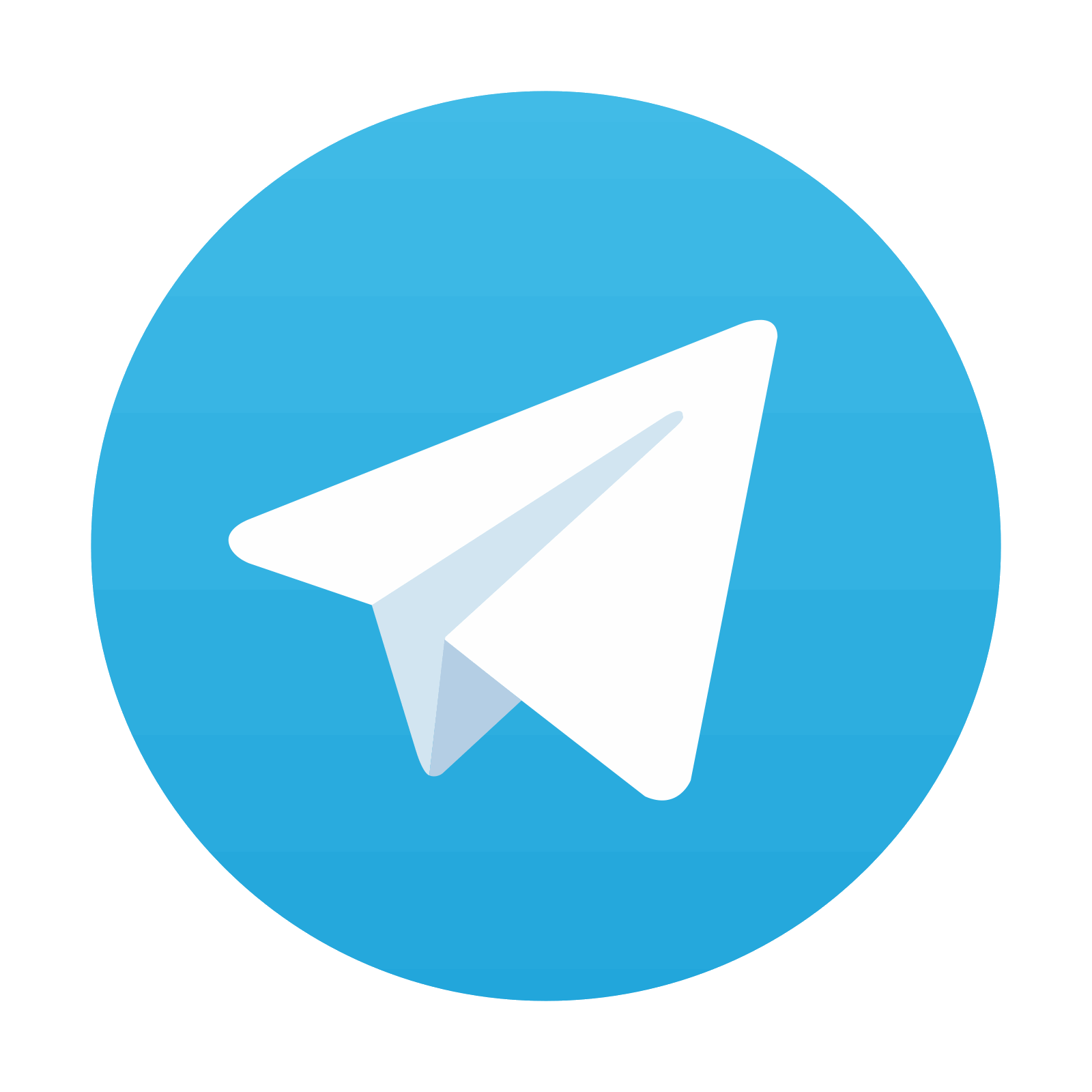
Stay updated, free articles. Join our Telegram channel
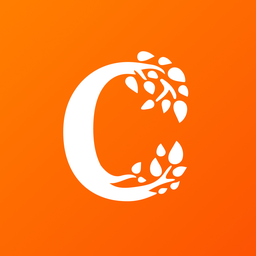
Full access? Get Clinical Tree
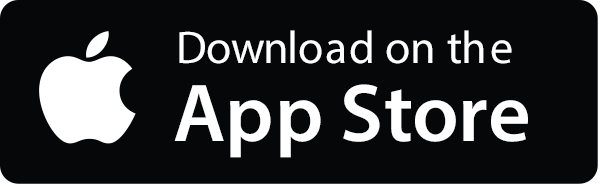
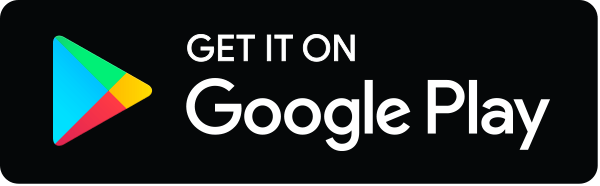