37 Fluid Flow and Strain in Bone
It has been well established that lack of physical exercise leads to bone loss, though this can be prevented by mechanical stimulation. Bone mass in athletes performing high-impact activities such as running, basketball, or tennis is much higher when compared to bone mass in sedentary people. 1 The process of bone remodeling allows bones to adapt their mass and structure to mechanical loading. 2 During bone remodeling, bone resorption by osteoclasts is followed by bone deposition by osteoblasts. 3 Current scientific insights strongly suggest that mechanical adaptation by osteoclasts and osteoblasts is orchestrated by osteocytes.2 The osteocyte is a stellate-shaped cell with numerous processes, embedded within the mineralized bone matrix. Osteocytes are connected to each other via their processes, forming a network through very thin canals, the canaliculi. Of all cells in mature bone, over 90% are osteocytes.2 In response to a mechanical stimulus, osteocytes transduce the mechanical signal into a biochemical signal (i.e., production of growth factors, cytokines, and reactive nitrogen and oxygen species). These signaling molecules activate osteoblasts to form new bone.2 In the absence of a mechanical stimulus, osteocytes produce molecules that stimulate osteoclast development and activity.2 Osteoblasts themselves are able to respond to mechanical loading as well. 4
Mechanical loading on bone causes tiny deformations in the stiff mineralized bone matrix. The cells that are attached to the deforming bone matrix will deform as well (Fig. 37.1). Quantitative studies on animal and human bones found maximal loading-induced strains (matrix deformation) of 0.2 to 0.3%. 5 These deformations are very small, and one can wonder whether they are sensed at all by the cells. However, because bone is not a homogenous material, the magnitude of global (end-toend) strain that is applied on bone will be different from the local strains within the bone matrix. 6 Strain distributions in bone are highly heterogeneous, and therefore the strains that osteocytes experience in vivo might be much higher than the applied strain to bone.

When bones are loaded, the resulting deformation will drive a thin layer of fluid through the canaliculi around the network of osteocytes.2 This can be compared with squeezing a stiff, water-soaked sponge. The fluid flows from regions under high pressure to regions under low pressure, and results in a fluid shear stress, ranging from 0.8 to 5 Pa. 7
In vitro studies have demonstrated that bone cells are sensitive to both fluid shear stress and tensile stress, and that these two types of mechanical stimuli have different effects on bone cells with respect to their biological response. 8 In the next paragraphs, two in vitro methods are described that can be used to study the effect of fluid flow and tensile strain on bone cells in a two-dimensional environment. Increasing our knowledge of how bone cells respond to mechanical loading in vitro will help to better understand the processes involved in the in vivo–induced adaptation of bone mass and structure in response to mechanical loading.
37.1 Mechanical Loading of Bone Cells in Vitro
37.1.1 Parallel-Plate Flow Chamber
The effect of physiological flow regimes on the biochemical response of bone cells can be studied using a parallel-plate fluid flow chamber (Fig. 37.2). A cell monolayer attached to a glass slide is subjected to fluid flow by forcing the medium to flow between two parallel plates, creating a pressure gradient along the chamber. The shear stress (τ) induced by a specific flow is mainly determined by the flow rate (Q) of the culture medium, the fluid viscosity (µ), the width (b) of the channel, and the height (h) difference between the two parallel plates: τwall=6μQbh2\tau _{{\rm{wall}}} = {{6\mu {\rm{Q}}} \over {{\rm{bh}}^2 }}. For details about the required dimensions of the chamber, and characterization of the chamber for dynamic fluid flow experiments, see Bacabac et al. 9

37.1.2 Membrane Stretch
The biochemical response of bone cells to strain can be investigated in vitro using a Flexcell Tension Plus System (Flexcell Corporation, McKeesport, PA). This system uses vacuum to deform a flexible-bottom culture plate (Fig. 37.3). The precision, accuracy, and repeatability of the strains controlled by the system, resulting in deformations to growing cells, are essential to analyze and interpret the results accurately. It is therefore recommended to validate the system before starting experiments (Note 37.1 (p. 297)).

37.2 Mechanical Loading of Bone Cells by Pulsatile Fluid Flow
Jargon Simplified: Pulsatile Fluid Flow
Mechanical loading of bone cells by pulsatile fluid flow results in a dynamic fluid shear stress onto the cells. This fluid shear stress mimics in vivo loading on bone cells that are located in the canalicular network of bone.
37.2.1 Materials Cell Culture Media and Solutions
Culture medium: alpha-Modified Eagle′s Medium (22571, GIBCO, Paisley, UK), supplemented with 10 µg/mL penicillin (Sigma-Aldrich, St. Louis, MO), 10 µg/mL streptomycin (Sigma-Aldrich), 50 µg/mL fungizone (GIBCO), and 10% serum (10% heat-inactivated fetal bovine serum [FBS] when using the MC3T3-E1 cell line; 5% FBS plus 5% heat-inactivated calf serum [CS] when using the MLO-Y4 cell line). When using other cell types, change the contents of the medium according to the requirements of that specific cell type.
Flow medium: alpha-Modified Eagle′s Medium supplemented with 10 µg/mL penicillin, 10 µg/mL streptomycin, 50 µg/mL fungizone, and 2% serum (2% FBS when using the MC3T3-E1 cell line; 1% FBS plus 1% CS when using MLO-Y4 cell line).
Sterile phosphate-buffered saline (PBS), pH 7.4.
Trypsin-tetra sodium ethylenediamine tetraacetic acid (EDTA) solution consisting of 0.25% trypsin and 1 mM EDTA 4Na.
70% ethanol.
Griess reagent for determination of nitric oxide (NO) production. Solution A: 2% sulfanylamide and 5% phosphate in water. Solution B: 0.2% naphtylethelene diamine HCl in water. Store the solutions separately in a refrigerator and protect from light.
0.1 M NaNO2 in water (stock solution) for quantification of NO production.
37.2.1.2. Instruments
75 cm2 tissue culture flasks
94/16 mm cellstar petri dishes
Fluid flow apparatus (Fig. 37.4):
Fig. 37.4 Fluid flow apparatus: A: Pump. B: Flow chamber. C: Glass slide with cells (only part of the slide containing the cells is exposed to fluid flow). D: Flow probe. E: Medium reservoir. F: Water bottle. G: Incubator.
37°C incubator
Water reservoir
Gas phase of 5% CO2 in air (or use CO2 independent medium)
Parallel-plate flow chamber (Fig. 37.2). The chamber provides a controlled laminar flow over the cells (Note 37.2 (p. 298)).
Poly-l-lysine-coated (50 µg/mL) or collagen-I–coated glass slides
Medium reservoir
Microannular gear pump (Mikrosysteme GmbH, Germany) (Note 37.3 (p. 298))
Flow probe
37.2.2 Methods
Make sure that all materials are sterilized before use. Work in a laminar flow cabinet as much as possible.
Cell Culture
Culture cells in T75 flasks in a humidified atmosphere of 5% CO2 in air at 37°C in culture medium, and refresh culture medium every 3 to 4 days. Trypsinize the cells upon 90% confluency:
Remove the culture medium and wash the cells in T75 flasks thoroughly with 5 mL PBS.
Remove PBS and add 1 mL of trypsin-EDTA at 37°C.
Incubate the cells in trypsin-EDTA up to 10 minutes at 37°C. Make sure that all cells are loose by tapping the flask gently on the side.
Add 8 mL of culture medium to the trypsin/cell suspension in the flask and transfer the cell suspension to a 15 mL plastic sterile tube.
Centrifuge the cell suspension at 1,000 g for 10 minutes.
Discard supernatant, resuspend cells in 1 mL culture medium, and count the cells with an automatic cell counter or a cell counting chamber.
Seed 2 × 105 cells in a new T75 flask to keep cells in culture, and culture until the cell layer reaches 90% confluence again, or seed the cells on a glass slide for treatment with fluid flow.
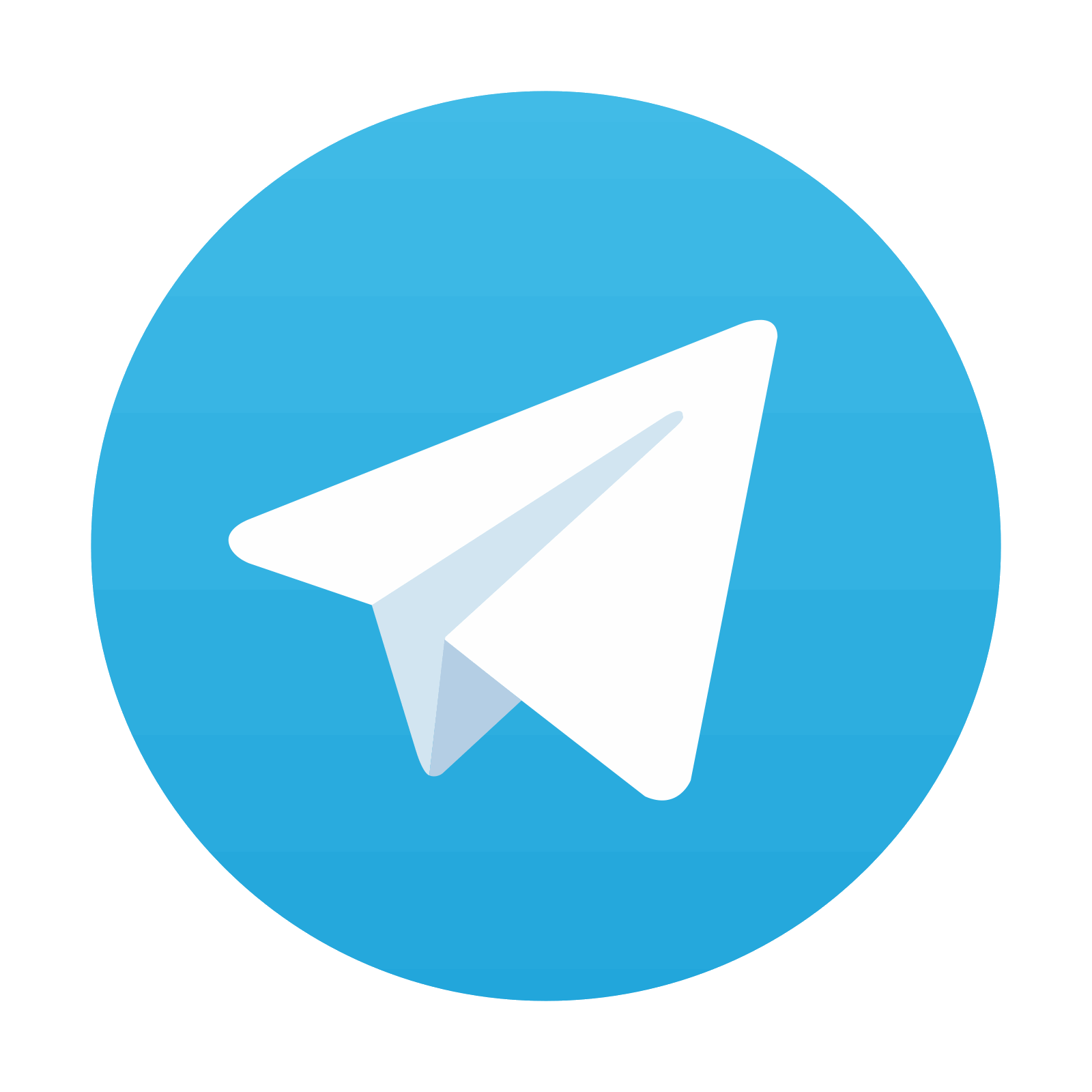
Stay updated, free articles. Join our Telegram channel
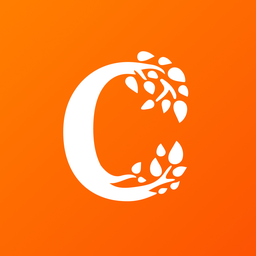
Full access? Get Clinical Tree
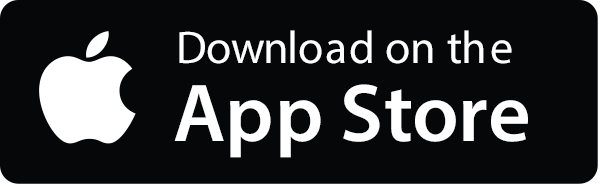
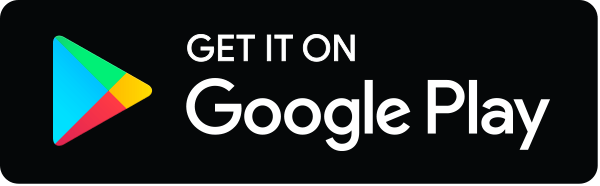
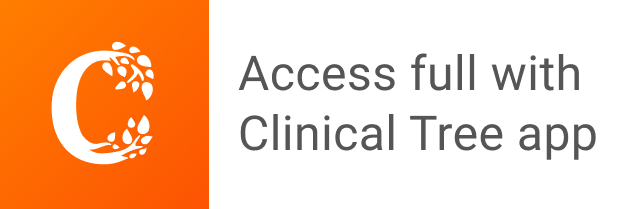