25 Micro-Computed Tomography Imaging of Bone Tissue
Since the introduction of micro-computed tomography (µCT) for imaging of trabecular bone approximately 25 years ago, this imaging modality has rapidly become the standard technique for assessment of bone micro-and macrostructure in laboratory research. Estimating bone mineral density from µCT images has also become commonplace. The purpose of this chapter is to provide an introduction and survey of the use of µCT in bone research. We begin with a brief overview of the basic operating principles of µCT systems and then proceed to summarize the capabilities of µCT as an imaging modality for assessing the microstructure, macrostructure, and mineralization of bone. The particular application of µCT to rodent bone is then discussed, along with applications that use µCT imaging to assess the biomechanical behavior of bone. We end the chapter with a summary of the recent trajectory of research in µCT imaging of bone.
25.1 Overview of Micro-Computed Tomography
Modern µCT systems are the evolutionary successors of X-ray technology, incorporating rapid digital image acquisition and high-performance computing to produce detailed, three-dimensional (3D) fields of data. All µCT scanners use the same basic operating scheme, which is to place the specimen in between an X-ray source and Xray detector, and to rotate either the specimen or the source-detector pair during the course of image acquisition (Fig. 25.1). The X-ray source produces photons, which are attenuated as they pass through the specimen, prior to striking the detector. The detector is an X-ray–sensitive camera that records a two-dimensional (2D) radiographic projection of the specimen at the particular angular position. The angular position is then changed, and a new projection is gathered. The number of projections required depends on the nature of the specimen and the desired image resolution, but usually several hundred projections (e.g., one per degree of rotation) are necessary. The resulting set of 2D projections is then reconstructed to generate image data representing the full 3D volume. This 3D representation is simply a 3D array of voxels (volumetric pixels).

For the typical range of photon energies used in µCT (20 to 100 keV), photon attenuation occurs via photoelectric absorption and Compton scattering. Photoelectric absorption, whereby the attenuation scales with the atomic number of the material, is foremost for lower-energy photons (< 25 keV). Compton scattering, whereby the attenuation scales with the density of the material, is dominant for higher-energy photons. Owing to its high calcium content and high density, bone tissue attenuates the incident photons to a greater degree than do soft tissues. This differential attenuation produces sharp contrast between bone and surrounding soft tissues in µCT images, wherein the grayscale value of a given voxel represents the attenuation of the material present at that location in the specimen.
Two different types of µCT scanners have been used extensively in bone research: X-ray tube µCT and synchrotron µCT. These two types of scanners differ in the nature of the C-ray source, availability, and imaging capabilities.
25.1.1 X-ray Tube Micro-Computed Tomography
X-ray tube µCT systems are so named because they use an X-ray tube to produce photons. They are generally known as “bench-top” µCT systems because of their comparatively small size. X-ray tubes are solid-state components and are based on mature, commercially available technology. This combination of size, reliability, and low maintenance makes X-ray tube µCT the most common µCT system for research applications. To a large extent, the performance of x-ray tube µCT scanners can be characterized with just a few parameters (Table 25.1).
While X-ray tubes are compact and relatively inexpensive, one important drawback is that they produce only polychromatic X-rays. The emitted X-ray beam is thus subject to beam hardening, a phenomenon in which the photons of lower energy are preferentially absorbed as they pass through the specimen, resulting in a “harder” beam, or a beam of higher mean energy. Image artifacts created by beam hardening can confound measurements of mineral density and can also blur distinctions between high- and low-attenuating materials, thereby decreasing the effective spatial resolution. The negative effects of beam hardening can be reduced by placing an aluminum filter in front of the X-ray source to “preharden” the beam 1 and/or by applying a correction during image reconstruction based on empirically derived calibration data. 2
X-ray tube µCT scanners specially designed for high-resolution imaging can achieve isotropic voxel sizes of side length ~2 µm, though most bench-top systems are limited to ~5 µm. The best achievable resolution, expressed in terms of voxel size, is typically in proportion to the specimen diameter. For medium- to high-attenuating materials such as bone, the signal-to-noise ratio is more than sufficient to discern the material from background noise. However, these systems cannot be used to distinguish among low attenuating materials, such as cartilage and many other soft tissues, without use of a contrast agent.
25.1.2 Synchrotron Micro-Computed Tomography
Synchrotron µCT systems produce photons from synchrotron particle accelerators, rather than X-ray tubes, and are thus not as widely available as X-ray tube systems. Synchrotron radiation has several advantages over radiation produced by an X-ray tube. First, the beam is monochromatic and is not subject to beam hardening. Second, the narrower spread of photon energy makes for improved signal-to-noise ratios, especially when imaging lower-attenuating materials. Third, the synchrotron can generate a parallel photon beam (whereas X-ray tube sources generate fan- or cone-shaped beams), which enables greater magnification and improved spatial resolution.
Synchrotron µCT systems can achieve submicron resolution. As with µCT systems using X-ray tubes, the best achievable resolution expressed in voxel size is proportional to specimen diameter. Further, the absence of beam-hardening artifacts allows accurate measurement of mineral density in bone tissue. 3
25.2 Micro-Computed Tomography Imaging of Trabecular Bone
The first published reports of the use of µCT in bone research were studies of trabecular bone. 4, 5 Prior to these reports, the gold standard for quantifying the microstructure of trabecular bone was to cut thin, serial sections of a specimen and to perform histomorphometric analyses of each section. µCT provided an alternative that is nondestructive, 3D, and, with the continued improvements in computing power, increasingly more time-efficient and less labor-intensive.
Critical to the validity of µCT analyses of trabecular microstructure are choices of several parameters involved in scanning and image processing. For scanning, the parameters are the nominal resolution of the scan or, equivalently, the voxel size in the resulting image; the tube voltage and current; the integration time (the amount of time the detector is active for each projection); and the frame averaging (the number of times each projection is repeated). The chosen resolution should balance the need for accurate measurement of small features within the specimen with eight-fold increase in dataset size caused by doubling the resolution. For a feature such as a trabecula to be detected, the voxel size must be no greater than one half the thickness of the feature; however, in practice, the voxel size should be smaller, particularly for the purposes of quantitative analyses of microstructure and density. The appropriate tube voltage for bone specimens is typically toward the high end of the voltage range offered by bench-top µCT systems (20 to 100 kVp), because specimens of higher density and/or larger diameter require higher beam energies. The tube current, integration time, and frame averaging collectively determine the number of photons collected by the detector, which is generally proportional to the signal-to-noise ratio for the scan.
Regarding image processing, the key parameters are those that control the noise filtering and the threshold. Filtering is essential for noise reduction prior to calculation of the microstructural parameters. Filtering involves smoothing the spatial variation of grayvalues within a small neighborhood of voxels. A Gaussian filter is most commonly used. Selecting a correct threshold is crucial, because the threshold defines which voxels are considered to contain bone tissue (Fig. 25.2). For trabecular bone, routine algorithms exist to identify an appropriate threshold for segmenting bone voxels from those containing marrow, other soft tissues, and background medium. 6, 7 However, the binary images resulting from applying the threshold should always be examined in conjunction with the original, grayscale images to check on the suitability of the threshold. The aforementioned algorithms may not produce good segmentation of the microstructure for a given combination of voxel size and other scanning parameters. Moreover, use of a single value for the threshold (a global threshold) for the entire image and for every experimental group may not be feasible for a given study.

25.2.1 Trabecular Microstructure
A slate of common metrics describing the microstructure of trabecular bone now exits (Table 25.2). Bone volume (BV) is defined as only the volume of the specimen (or the region of interest [ROI] within the specimen) that is occupied by bone. Commonly, BV is more meaningful when it is normalized by the total volume of the specimen (or ROI); the resulting metric, BV/total volume, is known as the bone volume fraction. The remaining metrics quantify the trabecular architecture, or the arrangement of BV in space. Trabecular thickness (Tb.Th*: The measures of trabecular thickness, separation, and number that are appended with an asterisk are determined directly from the 3D images of the trabecular structure without making any assumption about the type of structure. Traditional histomorphometric measurements [e.g., Tb.Th] assume a type of structure, such as rod-shaped or plate-shaped trabeculae, in order to calculate these architectural metrics. 9) is the mean thickness of the individual trabeculae, while trabecular separation (Tb.Sp*) is the mean distance between trabeculae. Trabecular number (Tb.N*) is the average number of trabeculae per unit length for an arbitrary line through the volume. The structure model index (SMI) is a quantitative measure of how “rod-like” or “plate-like” the trabeculae are. The structure model index values typically range from 0 (plate-like) to 3 (rod-like) but can also include 4 (sphere-like) and negative values (trabeculae with concave surfaces that nearly enclose marrow pores). 10 Connectivity density is defined as the number of connections between trabeculae per unit volume. Degree of anisotropy (DA) describes the extent of preferential alignment of the trabeculae. A DA value greater than 1 indicates that a preferential alignment, or anisotropy, exists (consider, e.g., the primary compressive group in the femoral head). The minimum value of DA, 1, indicates no preferential orientation (i.e., isotropy). Importantly, DA quantifies the extent of the preferential alignment, not the direction of that alignment. However, the same calculations used to determine DA also identify the direction.
25.2.2 Tissue Mineral Density
In the absence of beam hardening, or at least with sufficient correction for beam-hardening artifacts, the gray-values or values of linear attenuation contained in µCT images can be converted into values of mineral density. This conversion requires a standard curve that can be obtained from a scan of a calibration phantom—a set of standardized specimens of known mineral density. It is important to note that the resulting values of mineral density are not true density measures but rather estimates of the partial density (mass concentration) of a mineral solution that would have the same linear attenuation as the region of tissue imaged. For example, if the calibration phantom contains varying concentrations of hydroxyapatite, then the mineral densities obtained from conversion of the voxel grayvalues are in units of mass of hydroxyapatite per volume (e.g., mg hydroxyapatite/cm3). This conversion can be done for any collection of voxels in the image; however, a standard approach is to report the average mineral density for all voxels whose grayvalue exceeds the specified threshold. This average value is known as the tissue mineral density (TMD).
Values of TMD are sensitive to partial-volume effects. These effects arise from the discrete nature of the image data: Voxels at the surface of a trabecula, or any boundary between materials with different attenuation, are only partially filled with the higher attenuating material and therefore have a lower grayvalue than would be commensurate with the higher-attenuating material alone. A coarse image resolution will exacerbate partial-volume effects; however, these effects will always be present to some degree. Moreover, while partial-volume effects can degrade the accuracy of all measures of trabecular microstructure, they are more damaging to measurement of TMD. Many techniques used to measure TMD will account for partial-volume effects by “peeling” a set number of voxels away at any interface identified during segmentation. For trabecular bone, users should exercise caution, because if the image resolution is not sufficiently high, this peeling may leave too few trabeculae with meaningful volume left for calculation of TMD.
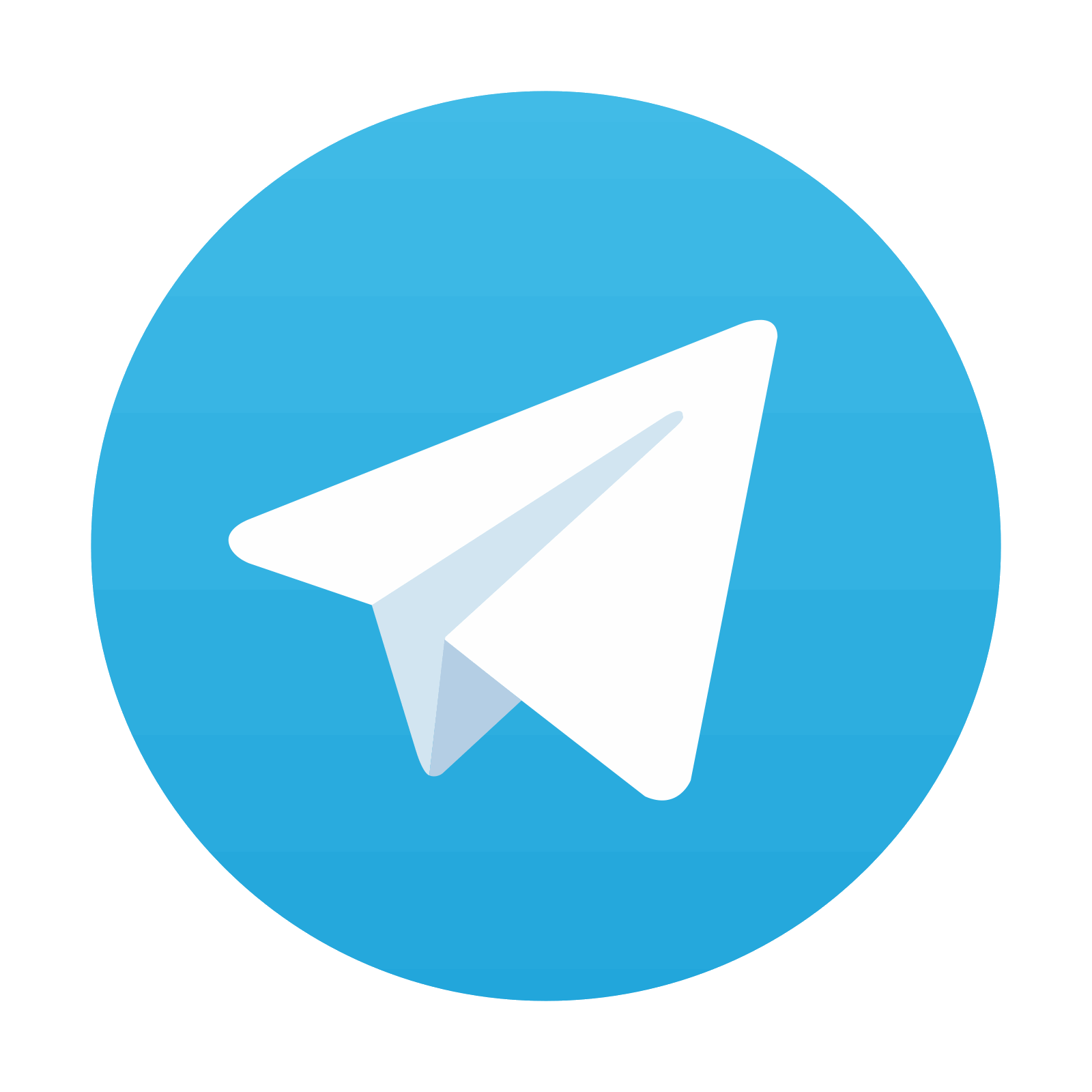
Stay updated, free articles. Join our Telegram channel
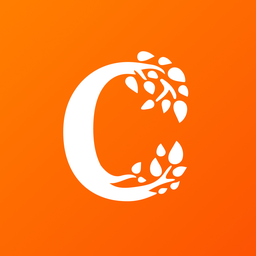
Full access? Get Clinical Tree
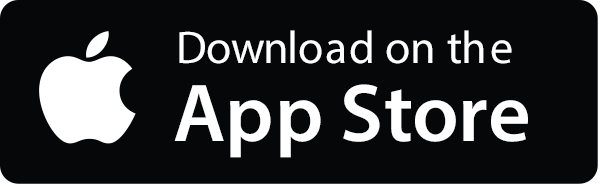
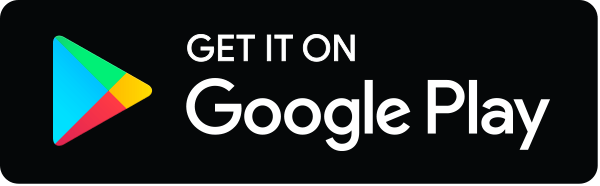
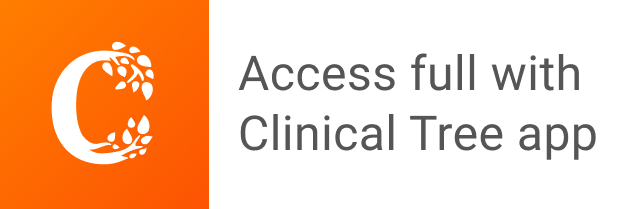