Abstract
Developments of hand-and-finger joint arthroplasty help to improve the quality of life for many patients suffering from rheumatoid arthritis, osteoarthritis, and posttraumatic conditions. Extensive research has been performed in the past few decades on biomaterials to replace damaged joints. Biomaterials are either used in their native form, altering their formulations, or combined with others materials such as for arthroplasties. We need to understand the mechanical properties of the biomaterials so that we can use the materials appropriately. The limitations of current materials and future research avenues will be discussed.
Key words
Biomaterials – biocompatibility – hand – polymers – silicone elastomers – metal alloys – calcium hydroxyapatite – pyrolytic carbon2 Biomaterials in Arthroplasty of the Hand
2.1 Introduction
Since 1890, when Themistocles Gluck performed the first wrist replacement using an ivory prosthesis, substantial effort has been directed onto the development of small joint prostheses for the hand. Success in arthroplasty relies upon a thorough understanding of joint mechanics, and implant design and materials employed for manufacturing the implant.
In 1969, Alfred Swanson defined the criteria for ideal joint arthroplasty (Table 2.1). He also pointed out that the materials used for manufacturing the implant component should be able to provide durable fixation to the host tissue and survive the stresses involved in joint movement. Moreover, the implant(s) should be biologically and mechanically acceptable to the host and should be easy to manufacture, sterile, and use. 1
Any material that has been engineered to interact with biological systems for a medical purpose is collectively known as biomaterials. According to the definition proposed by the European Society for Biomaterials Consensus Conference II, a biomaterial is “a material intended to interface with biological system to evaluate, augment, or replace any tissue, organ, or function of the body.” 2
I will provide an introduction to the basic concepts related to biomaterials science and review the main classes of biomaterials that are currently used in small joint and hand arthroplasty.
2.2 Behavior of Biomaterials
When an implant is inserted into the body, they are immersed into an environment that is more hostile than in air at room temperature. The higher temperature and sodium chloride content in the body as well as high stress concentrations lead to accelerated metal corrosion and degradation of polymers. At the same time, depending upon the types of material the human body will initiate a foreign body reaction. In order to allow the implant to perform the intended function for a sufficient period of time, the selection of biomaterials and their design must comply with the following basic requirements.
2.2.1 Biocompatibility
The definition of biocompatibility has been evolved over the years. In the 1960s and 1970s, the first-generation biomaterials tried to match the chemical and physical properties to those of the replaced tissue with a minimal host/foreign body response in order to minimize any biological rejection. 3 “Bioinertness“ was the underlying principle for these biomaterials and still holds true today for many implants.
With better understanding on the foreign body response in the 1980s and 1990s, there was a paradigm shift to develop bioactive components that can elicit biological bonding response at the bone–implant interface to improve implant fixation. 4 The ability to form an adherent interface with the living tissues has been defined as bioactivity. 5 Currently biocompatibility is the “ability of a material to perform with an appropriate host response in a specific application”; the “appropriate host response” refers to acceptable levels of toxicity and immune response, a lack of foreign body reactions, and promotion of normal healing. 6
2.2.2 Nontoxicity
Materials that are embedded into the body should not have any toxic effect due to release of ions or other harmful products that can develop unnecessary allergies, inflammatory, tissue necrosis, calcification, or even neoplastic hazards. 7 Examples of the failure of this are silicone synovitis 8 , 9 , 10 , 11 or metallosis in hip joint replacements. 12 , 13
2.2.3 Corrosion Resistance
Corrosion is unwanted degradation of metal immersed inside solution, which may result from electrochemical dissolution phenomenon, wear, or both. This property determines the metallic implant durability and should be very high if the implant is intended to be permanent. Passivation helps to protect metal from corrosion by forming a thin layer of oxide layer. 14
2.2.4 Strength
Strength describes the magnitude of stress at which the materials begin to fracture and correspond to implant durability. It depends on the type and vector of the force applied to the material. It can be described by multiple values, for example, compressive strength, tensile strength, and yield strength. 15
2.2.5 Modulus of Elasticity
The modulus of elasticity describes the ability of a material to undergo elastic deformation under stress. Table 2.2 provides the data of common biomaterials used in hand-and-finger joint arthroplasty. 16 , 17 , 18 A favorable value for implant anchorage or the intramedullary stem of an implant should be as close to that of cortical bone as possible. Otherwise, it will lead to stress shielding with bone resorption around the implant and the potential for bone–implant interface failure. 15 , 19
2.2.6 Fatigue Resistance
Fatigue is caused by a repetitive cyclic load below that of ultimate tensile strength of a material eventually causing deformations and fatigue fracture. For metallic biomaterials, this is strongly related to the type of processing and heat treatment used during manufacturing. 20
2.2.7 Wear Resistance
Low wear resistance is undesirable as it will produce wear particles into the articular or bone–implant interface, leading to an inflammatory reaction, osteolysis, and eventually implant loosening. Furthermore, corrosion will be accelerated and increase release of cytotoxic metal ions from worn implants. 21
2.2.8 Creep Resistance
Creep refers to plastic deformation of a material over a long period of time under constant pressure. High resistance to creep is important to decrease the deformation and wear of the articular component of arthroplasty. 22 Creep is a material feature of bone cement and used by some implants such as the Exeter stem to optimize outcomes.
2.2.9 Osteointegration
Osteointegration refers to a direct structural and functional connection between living bone and the surface of a load-carrying part of the implant. It may depend on the surface topography of the implant. 23 It can be critical for prosthesis longevity. Many methods of surface treatment have been developed to promote osteointegration, including trying to produce an optimal surface roughness or adding osteoconductive coating to promote bone ingrowth. 24
2.3 Classical Biomaterials
Four main types of biomaterials are currently used in clinical practice: polymers; metal alloys; ceramics; and pyrolytic carbon. Most of them were developed for the first generation of biomaterials typically developed from industrial purposes.
2.3.1 Polymers
Numerous classes of polymers have been used, e.g., silicone, polyethylene (PE), and polymethyl methacrylate (PMMA; bone cement).
Silicone
Swanson marked the start of modern era of small joint arthroplasty with the development of the silicon spacer in 1966. 1 , 25 26 , 27 Silicones also known as polysiloxanes are polymers composed of repeating siloxane units forming chains of alternating silicon and oxygen atoms (Fig. 2.1). Silicone elastomers are created by three-dimensional (3D) cross-linking of linear silicone polymer chains. Chemical inertness, heat stability, and durability have led to its widespread use in medicine. Good biotolerance, high hydrophobic capacity, and ability to withstand various sterilization process are further benefits allowing use of silicone elastomers in implant arthroplasty. 26 , 28 29 30 31 32 , 33

Hardening of silicone elastomers is by curing, a chemical process which adds curatives to induce polymer cross-linking. 29 Tensile strength is further enhanced by incorporation of “filler.” Silicone elastomers for medical applications normally utilize amorphous silica filler to reinforce the cross-linked matrix. While mixing the silica with the silicone polymers, a hydrogen bond is formed between hydroxyl groups on the filler’s surface and silicone polymer, resulting in higher tensile strength and better capacity to resist elongation (Fig. 2.1). 34
The original Swanson prosthesis was made from heat-vulcanized, medical-grade silicone elastomer stock. The prostheses are single-piece and formed in a mold at 121.1°C under 22.8 kg/cm2 of pressure. 1 Most silicone finger prostheses consist of intramedullary stems bridged by a hinge 26 (Fig. 2.2). The inherent flexibility of the silicone elastomer allows flexion and extension at the hinge and provides dampening effect on the bone. At the same time, it is stiff enough to maintain some joint alignment. The long-term functional stability of silicone prostheses occurs by development of well-encapsulated fibrous capsule which starts to develop within 3 days of implantation. 32

However, there are problems with the silicone elastomer implant. These include implant fracture, wear debris generation, and particulate synovitis.
Joyce et al analyzed the cause of silicone implant fracture by retrieving 12 Sutter metacarpophalangeal (MCP)prostheses from three patients. 35 They found that fractures generally occurred at the junction of the distal stem and hinge as a result of subluxing forces concentrated on MCP joint and flexion occurring at the stem rather than the hinge. In addition, the cortical bone of the proximal phalanx impinges on the dorsal surface of the distal stem of prosthesis. It is presumed that sharp spurs from the proximal phalangeal bone will produce small cuts over dorsal surface of the prostheses. With time, the crack propagates resulting in a fatigue fracture (Fig. 2.3). 35 , 36 Two-thirds of silicone MCP joint prostheses fracture by 14 to 17 years after implantation. 37 , 38 Fortunately, by the time a fracture occurs, a fibrous pseudojoint is formed. The finger joint has some stability and often do not require revision surgery. 26

During the process of abrasion and fatigue fracture, microscopic wear particles shed around the joint. This will stimulate proliferation and accumulation of mononuclear cells which will secrete cytokines and proteolytic enzymes, causing swelling and inflammation of pseudosynovial and synovial membranes. Persistent chronic synovitis can lead to fibrosis, osteolysis, and bone necrosis surrounding the prosthesis. Despite these downsides, silicone arthroplasty remains popular options in finger joint arthroplasty.
2.3.2 “Nouvelle Vague” for Hand Arthroplasty Development
Successes in total hip-and-knee arthroplasty were eye-catching. This led to the introduction of surface replacement into hand arthroplasty pioneered by Linscheid and Dobyns in 1979. 39
The concept was trying to reproduce a physiological articulation and retain the collateral ligaments to improve stability and in turn minimize osteolysis and subsidence. 40 There is no single biomaterial that can replicate all the biomechanical properties of different structures inside the joint. By using two or more biomaterials with different characteristics in various parts of implant, it can more closely match the natural material properties. This resulted in a new surface replacement design with a cobalt-chrome proximal component and an ultrahigh-molecular-weight polyethylene (UHMWPE) distal component for a proximal interphalangeal (PIP) joint surface replacement implant (Fig. 2.4). 39

Ultrahigh Molecular Weight Polyethylene (UHMWPE)
Polyethylene (PE) has the advantage of easily being formed into many different shapes. It is mainly used coupled with a metallic component. It is formed from ethylene gas and is polymerized by Ziegler–Natta catalyst (titanium(III) chloride) into UHMWPE powder. The powder is consolidated under high temperatures and pressure. Because of its high melt viscosity, the final product is produced by compression molding and ram extrusion. 41
UHMWPE is a high-density PE having a semicrystalline structure with a molecular mass more than 2,000,000 amu. The macromolecules consist of local ordered sheetlike crystalline lamellae embedded within amorphous regions and communicate with surrounding lamellae by tie molecules (Fig. 2.5). 42 It has been extensively used in large joint arthroplasty because of its low friction, high resistance to wear, and high toughness. 43

Medical-grade UHMWPE is free of calcium stearate and requirements are set according to the American Society for Testing and Materials (ASTM) 648–14. 44 Since the polymerization of UHMWPE requires a specialized production plant to handle the dangerous chemicals, there are only two companies capable of making these resins (Table 2.3). Despite identical manufacturing methods, there are slight variations in molecular weight and resin morphology which affects their mechanical properties and wear abrasion resistance. 45
The main factor responsible for UHMWPE failure in joint replacement is oxidative degradation. The presence of oxidation is related to the sterilization and storage methods. High-energy radiation such as gamma or electron beam radiation are the most commonly used sterilization methods for PE components. 46 A nominal dose of 25 to 40 kGy gamma radiation is commonly used, which will emit energy higher than that of the polymeric chemical bonds. This would generate the scission of some chemical bonds of the UHMWPE, and decrease its molecular mass. In the presence of oxygen, this will lead to the formation of free radicals, causing worsening of some chemical and physical material characteristics. 47 , 48 , 49 The oxidation process continues as long as there is an oxygen supply. This phenomenon is known as post-irradiation aging. It has been shown to occur in UHMWPE implants that were gamma sterilized in air and packaged in air-permeable packaging. 50 , 51 , 52 Decreased abrasive wear resistance, due to oxidation, leads to a decrease in mechanical properties such as abrasive wear resistance which results in the formation of wear debris and consequently osteolysis, which has been recognized as being the main cause of failure in orthopaedic implants. 53
To decrease the effect of oxidation on wear and the mechanical properties of UHMWPE, orthopaedic implant manufacturers modified their sterilization protocols aiming to reduce the amount of oxygen exposure during storage. These include gamma radiation sterilization in vacuum-packaging or inert-gas packaging. However, free radicals that are already present cannot be eliminated and in vivo oxidation is still possible. 54 Therefore, several methods have been attempted to improve the wear resistance of UHMWPE, including cross-linking, thermal treatment, and the addition of antioxidant vitamin E.
Cross-linking is achieved by formation of active sites at the chain ends, which can recombine to form trans-vinylene bonds. 55 This can be achieved by gamma radiation and the peroxide method. The wear rate of highly cross-linked UHMWPE is six times less than conventional UHMWPE. 56 These should allow manufacturers to produce thinner inserts that could be applied in hand arthroplasty. However, as the cross-linking density increases, elastic modulus, ultimate tensile strength, yield strength, and ductility reduce, which reflect the reason why cross-linked UHMWPE has less resistance to fatigue crack propagation. 56 Therefore, it is preferable to have optimal cross-linking density and keep it local to the surface to retain the bulk mechanical properties. 57
To remove free radicals formed during the cross-linking or sterilization of UHMWPE, thermal treatment has been employed. Two common thermal treatments are remelting and annealing. Remelting is when the temperature is elevated above the melting point (150°C), which allows free radicals trapped inside the crystalline region to diffuse out. Typically, the residual free radicals drop to undetectable level with this method. 54 But remelting has been shown to significantly reduce the degree of crystallinity, which affect the mechanical properties. 58 , 59 During annealing the temperature does not exceed the melting point, so the crystalline region does not undergo dissolution but this leaves measurable amounts of free radicals inside processed UHMWPE, which is still susceptive to in vivo oxidative degradation. 60 , 61
Vitamin E is a natural antioxidant, which is able to consume the free radicals and improve wear resistance. Two methods of incorporation are currently used: one is to blend vitamin E (concentration less than 0.3 wt.-%) with UHMWPE powder before consolidation; and the other is to allow vitamin E to diffuse into the bulk UHMWPE. Vitamin E–containing UHMWPE has shown superior oxidative stability with greater mechanical and fatigue strength; these are now used clinically. 62 , 63 , 64
Polymethylmethacrylate (PMMA)
Polymethylmethacrylate (PMMA) is commonly known as bone cement. It has been the material of choice for implant fixation to the host bone over 50 years, after being popularized by John Charnley in 1958. 65 The primary function of PMMA is transfer of weight-bearing forces from bonetoimplant and vice versa. The term “cement’ is a misnomer because PMMA has no adhesive properties. Rather, it acts as a space-filler to create a close mechanical interlock between the rough medullary canal and the implant. This close association leads to optimal stress and interface strain energy distribution which are paramount in longevity of the implant. If the extreme stresses generated exceed the capability of the bone cement to transfer and absorb forces, fatigue fracture will result.
PMMA is an acrylic polymer that is formed by mixing liquid methyl methacrylate (MMA) monomer and a powdered MMA-styrene co-polymer. Benzoyl peroxide (BPO) in polymer powder acts as initiator while N,N-dimethyl para-toluidine (DMPT) or dimethyl para-toluidine (DMpt) in liquid monomer act as an accelerator for the free-radical polymerization reaction at room temperature (cold curing). To avoid premature polymerization of the liquid component during storage, hydroquinone is added as stabilizer (Table 2.4).
Most of the commercial bone cements have a polymer powder/liquid monomer ratio of 2:1 (weight in g/volume in mL) in order to decrease the shrinkage level due to the density change in MMA-to-PMMA conversion during the polymerization. When mixed together, the reaction starts to take place between BPO initiator and the DMPT accelerator forming benzoyl free radicals at room temperature. These unstable free radicals start the initiation step of polymerization by forming a new chemical bond between the initiator fragment and one of the double carbon bonds of the monomer molecule. A new free radical is created on the activated monomer molecule which can react with the double carbon bond of a new monomer molecule in the same way as the initiator fragment did. This process, known as propagation, takes place over and over again to form a long chain of monomeric units. Polymerization ceases when there is regrouping of the two radical chains leading to depletion of free radicals (Fig. 2.6). 66

The free-radical polymerization process of PMMA bone cement is a highly exothermic chemical reaction as a result of energy released from the breaking down of C=C double bonds during polymer chain propagation. The invitro peak temperature can reach 110°C. It is particularly influenced by the liquid monomer composition, the polymer powder/liquid monomer ratio, and the radio-opacifier content. 67 Bone necrosis can occur when exposed to temperature of 50°C for 1 minute or 47°C for 5 minutes. 68 Although clinical studies have showed lower peak temperatures (40–47°C) has detected at the bone interface, which may be due to a thinner cement coating and heat dissipation through the broad implant surface and local blood circulation. 69 , 70 However, thermal-induced bone necrosis has been shown in animal model study and the possibility of leading to early loosening and subsequent implant failure are still the concern. 71
Various additives improve the workability of the polymer (Table 2.4). To make the cement radiopaque, contrast agents such as barium sulfate (BaSO4) or zirconia dioxide (ZrO2) are added. Chlorophyll dye helps to distinguish bone cement from native bone and facilitate removal when performed revision surgery. Antibiotic-loaded bone cements are often used as drug-delivery systems. Implants are more susceptible to bacterial colonization on their surfaces because the bacteria can form a glycocalyx-blocking immune scrutiny by the body and leading to periprosthetic infection. The local active antibiotic levels are often not sufficient to reach a therapeutic dose when delivered by the intravenous route alone. When loaded with antibiotics, bone cement functions as carrier matrix and offers a synergistic effect with systemic antibiotics. 72 Many antibiotics has been added to PMMA bone cement clinically but many factors including bacteriologic, physical, and chemical aspects need be assessed to optimize outcomes (Table 2.5). 73
During different stages of PMMA bone cement polymerization, the appearance and handling characteristics change. There are four different phases. The mixing phase is the period when thorough mixing of the powder and liquid component takes place. It can be mixed manually or with a commercially available mixing system with the ability to apply a vacuum to minimize the number of pores; this phase lasts around 1 minute. In the next (waiting) phase the viscosity of bone cement progressively increases to a condition that can be handled without sticking to surgical gloves; it lasts for several minutes. The bone cement surface often shows the distinctive change from a gloss to a matt appearance. The working phase is the period during which the surgeon can apply the cement and insert the prosthesis; this lasts around 2 to 4 minutes. The viscosity should be high enough to withstand the bleeding pressure but fluid enough to allow cement interdigitation into the surrounding cancellous bone. These two phases often depend on the type of cement and the handling temperature. Bone cement hardens when reaching the setting phase; this is the exothermic period with peak temperatures. The overall setting time, starts from the time of mixing liquid and powder to the time when the cement temperature drops to halfway between the ambient and maximum temperature; it usually lasts 8 to 10 minutes. Many factors can affect the setting time (Table 2.6). 74 , 75 , 76
Some of the designs of MCP and PIP joint surface replacement require cement to help to accommodate the variations in endosteal canal configuration and to restore proper alignment. 40 PMMA bone cement serves as a suitable material to fill up the defect between the implant and bone because of its excellent intraoperative workability. However, hand joint arthroplasty systems do not have any cement restrictor or cement gun to achieve pressurization, meaning there will be a less uniform and so weaker cement mantle that is prone to developing overstress. This may lead to cement fracture with release of cement particles which would induce a local inflammatory reaction and possibly periprosthetic osteolysis. 77
Metals Alloys
Metals alloys are constituted from metallic and nonmetallic elements which are frequently used because of their mechanical strength. Most of the metals used for biomedical applications comply with the ISO 5832 standard. They mainly include three families of metals: stainless steel; cobalt chromium (CoCr) alloys; and titanium (Ti) alloys. 19 They have different mechanical properties and corrosion responses. All metal alloys are stiff, ductile, and hard. Appropriate metal selection for a specific application requires consideration of a variety of parameters.
Pure metal forms a crystalline lattice microstructure, i.e., a repeating 3D unit with a closely packed, high-density arrangement of metal atoms. They are packed into one of three crystalline arrangements: body-centered cubic, face-centered cubic, or hexagonal close packed (Fig. 2.7). However, the units often do not combine perfectly and form clusters of grains, imprecisely aligned with each other. This causes imperfections within grain microstructure and defects in macrostructure known as scratches and voids. The size of the grains affects the mechanical properties of strength and isotropy on the materials. Biomechanically the larger the grain size, the earlier material fatigue and failure. 14

Alloys are usually formed by adding the alloying metal elements to the principalmetal element in the molten state. When it solidifies, the metallic alloying elements, such as chromium and nickel in stainless steel, substitute for iron atoms in the crystalline lattice because of similar atomic sizes. When nonmetallic alloying elements are added, they are smaller and so fit in the spaces among the metal atoms. Deformation of the crystalline lattice occurs, which, in turn, causes resistance to the flow between crystal units leading to increase in resistance to plastic deformation and yield stress. 14
Several steps are required to turn raw metal alloys into metallic components for joint prostheses. Casting is regularly used in manufacturing orthopaedic implants as it provides an efficient way for mass production that is difficult to achieve by machining. But the casting process produces large grains and metallurgical imperfections so they have lower mechanical properties than wrought or forged alloys. Forging is a manufacturing process to optimize material properties under pressure and classified as cold or hot working relative to its recrystallization temperature. Cold working increases the number and density of strains by plastic deformation at room temperature which makes it difficult to deform further. The yield strength increases but ductility decreases. Hot working is plastic deformation above the recrystallization temperature; it allows an increase in grain size which increases homogeneity and ductility. Sintering is the process of compacting and forming a solid mass of material by heat or pressure without reaching the melting point of the material. It can increase the mechanical strength of the material and is used for the shaping process of materials that have extremely high temperature. A summary of different techniques producing the metallic parts of joint prostheses from various alloys are listed in Table 2.7. 14 , 78 79 80
Stainless Steel
Stainless steel was the first metal alloy used for orthopaedic implants. It normally comprises carbon and iron. If chromium is added, it will form a corrosion-resistant coating of oxide of CrO3. 80 AISI 316L is the mostly commonly used stainless steel in orthopaedics. The number 316 stands for 3% molybdenum and 16% of nickel added into the alloy and the letter “L” refers to low carbon content (less than 0.03%).The low carbon content improves corrosion resistance as carbon reacts with chromium to form brittle carbides, which exposes the metal to corrosion and subsequent failure. 14 Its superior fatigue strength at a relatively low cost and easy processing means it is used in many orthopaedic implants, e.g., plates and screws in trauma surgery. AISI 316L stainless steel had relatively good corrosion resistance but when compared with CoCr and Ti alloys, it still has the disadvantage of being susceptible to crevice and pit corrosion. In addition, stainless steel has 200GPa modules of elasticity, which is ten times stiffer than cortical bone. This modulus mismatch leads to peri-implant bone resorption and weakening of the implant bone interface, a phenomenon called stress shielding. 13 , 81 , 82 Currently, stainless steel is out of favor in manufacturing metallic components in joint arthroplasties.
Titanium Alloys
Titanium alloys were originally used in aeronautics and are now widely used in orthopaedic implants. Titanium 6Al4V is the most commonly used titanium alloy in orthopaedics. The number 6Al4V refers to the proportions of the alloying elements: aluminum (6%) and vanadium (4%). It has excellent corrosive resistance as it forms an oxide layer (TiO2) on the surface by passivation. The modulus of elasticity is about 50% of stainless steel, making it less rigid so lessening the effect of stress shielding. 14 Moreover, titanium implants can osteointegrate into bone, which greatly increases the biocompatibility and long-term survival of the implant device. 83 However, titanium has the disadvantage of notch sensitivity, i.e., scratches or notching of the surface can lead to fatigue failure. It is also less resistant to particle-induced wear and cytotoxic vanadium ion release occurs; hence, titanium alloy cannot be used as a bearing surface. 80 , 84
Cobalt Chrome Alloys
Cobalt chrome (CoCr) alloys contain cobalt (30–60%), significant amounts of chromium (20–30%) to improve the corrosion resistance, minor amounts of molybdenum which can decrease grain size in order to improve mechanical strength, as well as some carbon and nickel. 14 , 79 CoCr alloys exhibit excellent corrosion resistance, higher stiffness due to a higher modulus of elasticity (Table 2.2), and good wear resistance which is suitable for manufacturing joint bearing surfaces as well as the stem of the prosthesis. However, on direct contact with bone, the CoCr implant will take up most of the load due to their high modulus of elasticity; the stress shielding effect will lead to bone resorption and failure of implant fixation. 83 , 84 In order to overcome the mismatch in modulus of elasticity between the alloy and cortical bone, PMMA bone cement is used to fix the CoCr implant to bone since the modulus of elasticity of PMMA bone cement is lower than that of CoCr alloy. Nonetheless there are problems with long-term implant stability as no bone ingrowth occurs onto the implant and loosening can occur when the cement mantle breaks down. In the view of this, cementless options have been developed that require a special surface treatment for the metallic implant surface.
2.3.3 Surface Treatment to Improve Bone Bonding
The need to improve the rate and area of cementless implant osteointegration has led to development of surface treatments. Two methods had been developed: porous coating and osteoconductive coating.
Application of porous coating onto cementless implants was introduced in the 1970s and may give better results than cemented implants due to superior osteophilicity and the osteointegration which leads to bone ingrowth onto the coated implants. 85 , 86 Based on the experiences borrowed from total hip-and-knee arthroplasty, there are several established methods to produce porous metallic coatings (Table 2.8).
The roughness of the implant surface affects osteoblast adhesion and differentiation. Osteoblast-like cells grown on rough titanium surfaces show reduced proliferation and enhanced osteogenic differentiation with upregulation of alkaline phosphatase (ALP) activity and the osteogenic differentiation marker osteocalcin. 87 , 88 , 89 , 90 , 91 , 92 , 93 This is likely to be mediated by integrin α2β1 with upregulation of a range of osteogenic growth factors including transforming growth factor 1 (TGF-1), prostaglandin E2 (PGE2), Wnt pathway agonist Dickkopf-related protein 2 (Dkk 2), vascular endothelial growth factor (VEGF), epidermal growth factor (EGF), and fibroblast growth factor (FGF). 93 , 94 , 95 , 96 In addition to their effect on osteoblasts, microrough surfaces also inhibit osteoclast activity by upregulating receptor activator of nuclear factor kappa-B ligand (RANKL) and decoy receptor osteoprotegerin (OPG) on osteoblasts which indirectly promote net bone deposition. 92 , 97
Surface porosity allows direct osteogenic cells ingrowth onto the implant, strengthening the bone–implant interface. A number of research groups have investigated the effect of pore morphology and dimension, showing that pore size (50–400μm), pore interconnectivity (75–100μm), particle interconnectivity, volume fraction porosity (3–40% for spherical beads), and the percentage area coated are factors that are important for porous coating fixation. 79
Another method to promote osteointegration is to introduce osteoconductive materials onto the implants. This has developed around second-generation biomaterials. Bioactive materials have been added to uncemented components to enhance the fixation; calcium hydroxyapatite (HA) is the most popular additive biomaterial.
Calcium hydroxyapatite (HA)
Calcium hydroxyapatite is a ceramic. Ceramics are compounds of metallic elements bound ionically and covalently with nonmetallic elements. The calcium-to-phosphate ratio of HA resembles the minimal phase of bone, which accounts for their osteoconductive features. 98 Once HA is implanted in vivo, a layer of carbonate-hydroxyapatite (CO-HA) will develop on its surface as a result of ion exchange with the surroundings. 99 , 100 , 101 , 102 The resulting coating layer on the HA is known as carbonate-hydroxyapatite (CO-HA); it resembles the apatite present in normal bone. 103 The new apatite layer acts as a scaffold for osteoblasts and with time, it is further resorbed by osteoclasts and replaced by new bone. 101 Some studies have shown that HA-coated implants reduce the migration of components and have higher survival rate of comparable press-fit implants. 104 However, because of its low interface bonding strength and low toughness of the HA layer, it can result in fracture and delamination from the stem surface leading to osteolysis or third-body wear if the debris migrate into the joint space. 105 A different coating technique has been investigated with the aim to improve the interface bonding strength of the HA layer (Table 2.8). 79
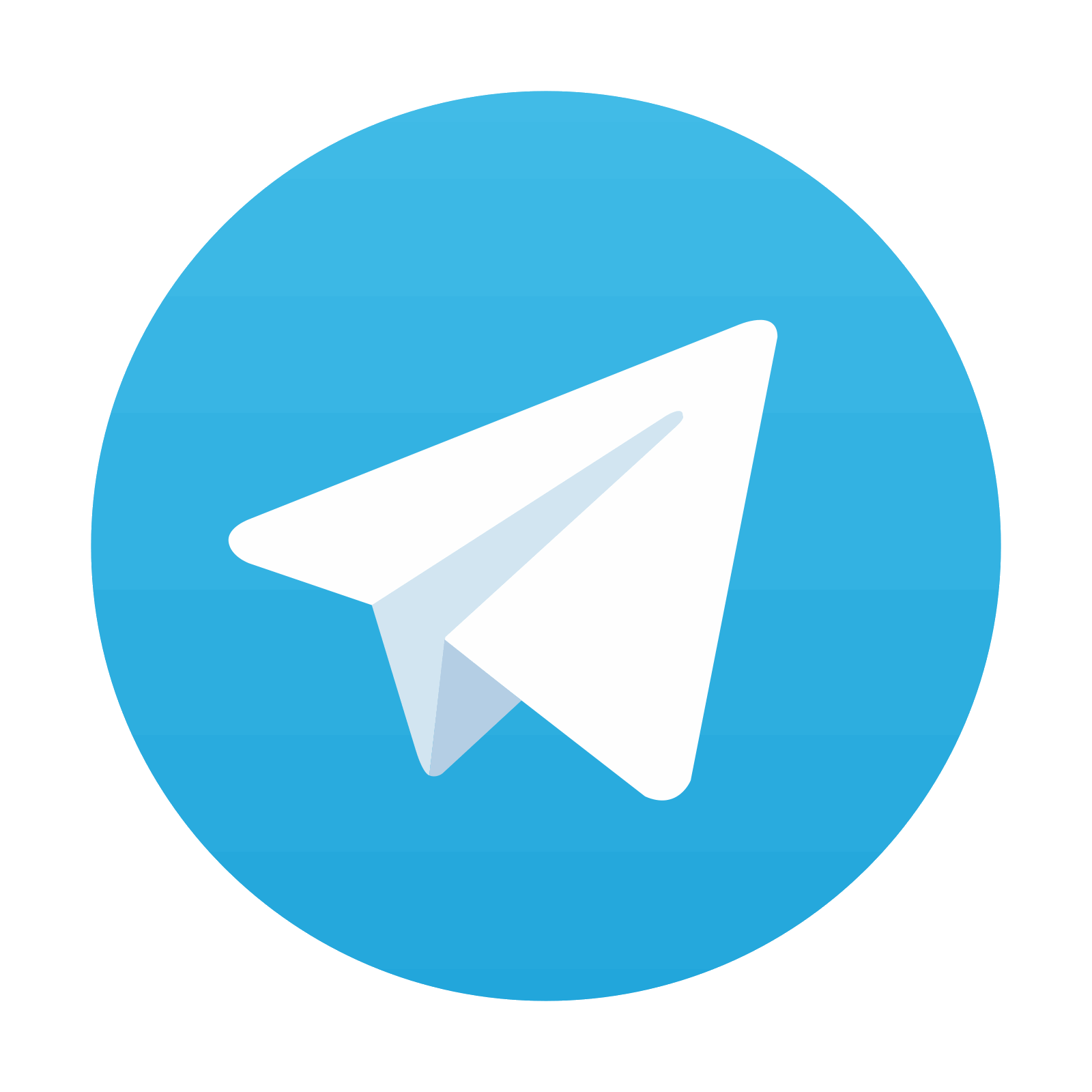
Stay updated, free articles. Join our Telegram channel
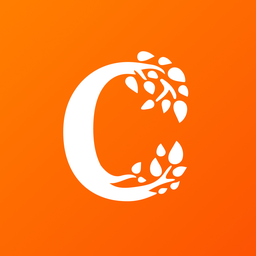
Full access? Get Clinical Tree
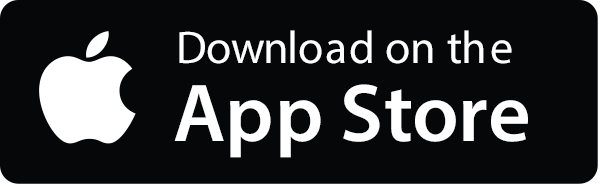
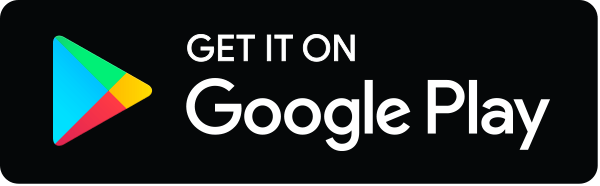
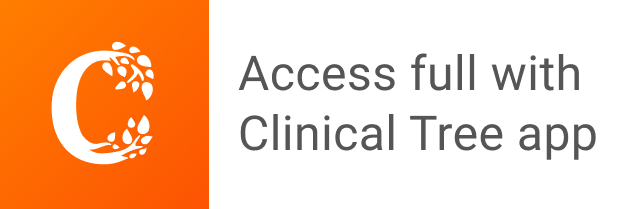