2.2 Biomechanics of acetabular fractures
1 Normal hip mechanics
The hip joint appears to be a simple ball-and-socket articulation. However, the joint is complex in both the forces crossing the joint as well as the distribution of forces within it. Mechanical forces acting across the hip joint are complex and not easily quantified precisely. Forces across the joint itself are greatest during mid-stance and are derived from two primary sources: body weight (BW) and abductor moment. During single-leg stance, these two forces reach equilibrium. The abductor force (ABD) is greater than BW owing to a shorter moment arm, so that in the steady state (BW × a) = (ABD × b) ( Fig 2.2-1 ). The joint reactive force (JRF) represents the sum of mechanical forces acting across the hip joint and is also expressed as a vector on the free body diagram. The JRF during stance when walking on level ground averages about 2.5−2.8 × BW and about 0.1−0.5 × BW during swing phase [1−3]. This value increases to 4.8−5.5 × BW when jogging, and almost 8 × BW with inadvertent stumbling. However, even sedentary activities generate significant forces across the joint with straight-leg raise 1.0−1.8 × BW and bed-to-chair transfer 0.8−1.2 × BW [1−3].

Although JRF is a calculated figure based on the sum of forces acting across the hip, contact pressures within the joint can be measured directly. During “normal” gait, contact pressures within the hip joint average 3−5 MPa during stance and about 0.5 MPa during swing phase with peak pressures of 5−10 MPa. When rising from a chair, peak stresses increase approximately threefold in the posterior acetabulum to 18 MPa secondary to muscle activation [1−3].
Several studies have revealed that the contact area within the hip joint appears to be load dependent. Dye transfer and dye exclusion techniques within the joint have shown increasing contact between the femoral head and acetabulum with increasing loads [4, 5]. However, other investigations [6−8] have demonstrated loading of the hip joint almost exclusively in the superior region, even at low loads. These reports typically use a model that explants the acetabulum from the pelvic ring and rigidly puts it on a loading jig.
In the mid-1990s Olson et al [9, 10] developed a methodology for testing the human hip of an intact pelvis using a simulated abductor mechanism to load the joint. Their method resulted in a distinctly different pattern of loading within the intact hip joint compared with those described in the literature. Other authors [5−8] loaded an acetabulum that was sectioned from an intact innominate bone. Bay et al [11] explored this question by comparing the load distribution of hips that were tested with two different mechanisms on the same day. They compared load across the hip of an intact pelvis via a simulated abductor mechanism to the same hip with the acetabulum explanted from innominate bone and loaded along the joint reaction force vector. The findings illustrate two distinctly different patterns of load distribution within the same hip resulting from different methods of loading the joint. These results strongly imply that explanting the acetabulum alters the relatively uniform-loading distribution of an intact pelvis loaded via an abductor mechanism to one of superior acetabulum (dome) loading with little anterior or posterior contact ( Fig 2.2-2 ) [11]. This supports the theory of deformation of the acetabulum about the femoral head under load in an intact pelvis. The loading pattern that results from deformation is lost with explanting of the acetabulum or with any fracture of the acetabulum that was tested [9, 11]. This same finding is observed in finite element models of hip joint loading that assume no deformation of the acetabulum.
The role of the acetabular labrum is of increasing interest. Many authors [12−15] believe the labrum in the hip may play a role in enhancing hip stability. These investigations use either an explanted acetabulum or a rigid body finite element model in their methodology [12, 13]. Konrath et al [16] demonstrated excision of the labrum resulted in no changes on intraarticular-loading characteristics within the intact hip in single-leg stance.
2 Acetabular fractures
The pathogenesis of posttraumatic degenerative arthritis has not been clearly elucidated, although several theories have been proposed. Clinically, there is a strong correlation between articular malreduction and accelerated degenerative changes [17]. Damage to the articular surface at the time of injury is difficult to quantify but nevertheless may be significant in joint pathogenesis. It is unclear what factors predominate in developing arthritis but undoubtedly bio-mechanical alterations in the joint are a major component. Various studies have focused on the biomechanical consequences of acetabular fractures. These studies can be divided into three equivalent categories, focusing on: (1) intraarticular contact area and pressure; (2) loss of congruence or instability after fracture; and (3) stiffness of fracture fixation.

2.1 Intraarticular load characteristics of acetabular fractures
Studies focusing on contact area and pressures argue that increased stresses within the cartilage exceed the capacity of the tissue to adapt, initiating a cascade of degenerative changes leading to joint arthritis. There is clinical evidence that increased peak pressures, especially in the superior region of the acetabulum, lead to degenerative arthrosis [18]. Several studies have focused on the alteration of contact area and stresses in the joint that occur as a result of fracture. These investigations were conducted with a model simulating the abductor mechanism using cadaveric pelvis with Fuji prescale film to record the intraarticular stresses. Common to all fracture patterns studied, including posterior wall, anterior wall, anterior column, and transverse, was the change from a uniform contact pattern to one of increased contact area and peak pressures in the superior acetabulum [10, 19−22].
Fractures of the posterior wall resulted in increased peak pressure and contact area in the superior aspect of the acetabulum with concomitant decreased pressures and contact area in the anteroposterior walls ( Fig 2.2-3 ). These changes were not reversed by anatomical reduction and rigid fixation using interfragmentary lag screws and a posterior wall buttress plate [10]. Varying the size of the posterior wall fragment altered contact area proportionally; however, the greatest change in contact area was seen in the smallest defects [22]. Olson et al [23] used a calcium phosphate cement to stabilize the posterior wall after fracture returned the joint-loading parameters toward the intact pattern of loading.
Investigations of a high-anterior column fracture demonstrated increased peak contact pressures with both step (10 MPa) and gap (12 MPa) malreductions [21]. Fractures of a low anterior wall fracture corresponding to a 45° roof arc measurement had no effect on peak pressure or contact area within the superior acetabulum [20].
Juxtatectal transverse fractures with step or gap malreduction (computed tomography [CT] subchondral arc of 9 mm) did not produce significant changes in peak contact pressures [19]. However, transtectal transverse fractures (CT subchondral arc of 1 mm) produced an increased peak contact pressure (20 MPa) in fractures with step malreductions. Increased peak pressures in the superior acetabulum with step malreductions of transtectal fractures also have been reported with an explanted acetabulum model. All these changes produced increased peak pressures in excess of 10 MPa. These findings mirror results of large clinical series of operatively treated acetabular fractures.

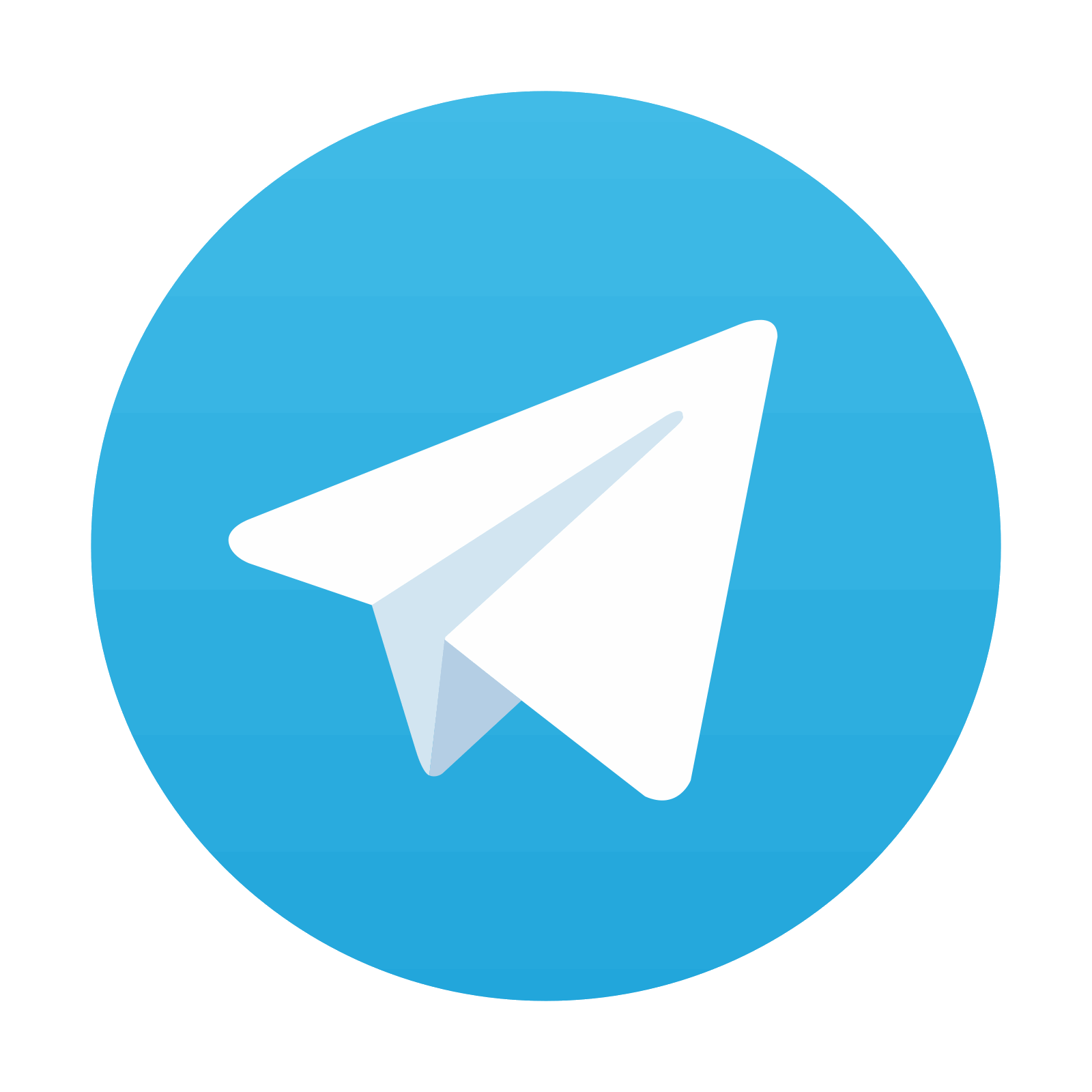
Stay updated, free articles. Join our Telegram channel
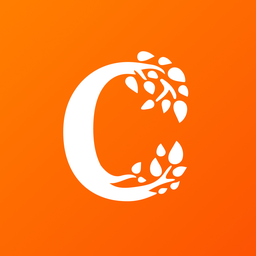
Full access? Get Clinical Tree
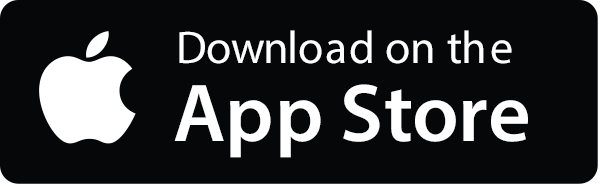
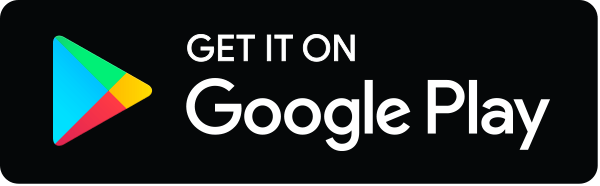
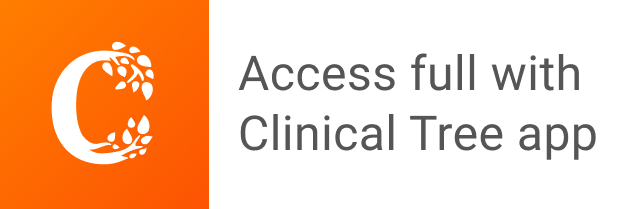