INTRODUCTION
Neurophysiologic studies can play a significant role in the assessment of peripheral nervous system disorders and suspected neuromuscular dysfunction. Neurophysiologic evaluation provides objective information about the diagnosis, localization, nature, and severity of the pathologic processes involved. Information obtained about the peripheral neuromuscular system can be used to plan and prescribe a therapeutic program. It also provides information on prognosis and the effectiveness of a treatment program. This chapter reviews basic principles of electrodiagnostic studies and the technical aspects of obtaining biologic signals from study subjects.
NEUROMUSCULAR ANATOMY & PHYSIOLOGY
The peripheral nervous system consists of the nerve root, the motor efferent from the anterior horn cell, and the afferent sensory root with dorsal root ganglion, usually located in the neural foramina—both of which combine to form a mixed spinal nerve. The mixed spinal nerve splits into the ventral and dorsal rami. The ventral rami from these nerves coalesce to form trunks, divisions, and cords, which ultimately become terminal nerve branches of the brachial plexus (to innervate the upper limbs) and the lumbosacral plexus (to innervate the lower limbs). The dorsal rami of the spinal nerves innervate the paraspinal muscles (Figure 16–1).
Nerve axons have electrical properties common to all excitable cells. The axons not only conduct the propagating electrical potential but also transport nutritional and trophic substances, the latter maintaining the metabolic integrity of the peripheral nerve. There is extracellular preponderance of sodium (Na) ions and intracellular preponderance of potassium (K) ions. At rest an excess positive charge outside the cell and negative charge inside the cell is maintained as a resting transmembrane potential by the cell wall. Voltage-gated sodium (Na+) and potassium (K+) channels are present in the cell wall. The density of the Na channels is high at the nodes of Ranvier in the myelinated axons. The influx of Na with efflux of K that occurs upon opening of these channels produces the action potential that is propagated along the axon. When a weak current is applied to a nerve, negative charges from the negative pole (cathode) make the inside of the cell relatively more positive, causing depolarization. After 10–30 mV of depolarization, the membrane potential reaches a critical threshold for generation of an action potential, which is bidirectional.
Motor action potentials that are generated at the cortical level travel through the brainstem and spinal cord to the muscle. At the level of the muscle the action potentials traverse the neuromuscular junction. The neuromuscular junction is a simple relay between the nerve terminals of a motor neuron and the skeletal muscle fibers innervated by that nerve. The electrical potential that arrives at the nerve terminal is converted at the neuromuscular junction to a chemical signal. The neuromuscular junction contains the presynaptic nerve terminal, a specialized postsynaptic muscle membrane, and the endplate, which underlies the nerve terminal (Figure 16–2).
Chemical transmission across the synaptic cleft is made possible by acetylcholine (ACh), which is synthesized from acetyl coenzyme-A and choline by the enzyme choline acetyltransferase in the nerve terminal. ACh is present in three compartments in the presynaptic terminal. The largest compartment is a reserve of ACh, or main store, with 300,000 quanta available. A smaller compartment, which serves as a “mobilization store,” is adjacent to the endplate zone with approximately 10,000 quanta of ACh available for movement to the nerve terminal. The smaller synaptic vesicles loaded with ACh aggregate near discrete areas of the presynaptic membrane in active zones, available for immediate release. In the resting state, a small quantity of ACh is released intermittently; this release is dependent on intracellular calcium (Ca) concentration that allows for binding of the ACh molecule to the postsynaptic membrane. Once released, the ACh diffuses across the synaptic cleft and binds to ACh receptors (AChR), which are concentrated on the postsynaptic folds.
Binding of ACh to its receptor causes the ion channel in the center of the AChR to open, resulting in a flux of Na, which depolarizes the muscle membrane at the junctional region. If only a single quantum of ACh is released, a nonpropagating depolarization is produced, resulting in a miniature endplate potential. The action of ACh is quickly terminated by the release of acetylcholinesterase, which breaks the molecule down into acetic acid and choline.
In contrast to intermittent random release of quanta of ACh at rest, there is coordinated release of up to 100 quanta of ACh into the primary synaptic cleft when an action potential is generated from the motor axon. As the action potential travels, depolarizing the presynaptic terminal, the voltage-gated Ca channels open, allowing Ca to diffuse across the presynaptic terminal. This triggers a coordinated movement of ACh vesicles toward the synaptic membrane, resulting in fusion and release of ACh to the synaptic cleft. This much larger release of ACh diffuses across the synaptic cleft, binding to a large number of ACh receptors and causing a larger nonpropagating depolarization of the postsynaptic membrane. This nonpropagating potential is called the endplate potential.
If this potential reaches the critical threshold for depolarization, an action potential is propagated. This action potential invades the tubule system of the muscle, resulting in release of Ca from sarcoplasmic reticulum and triggering muscle fiber contraction in all the muscle fibers innervated by the axon. Coordinated generation of a large number of motor unit action potentials is needed to contract the muscle. The larger a muscle is, the more motor unit action potentials are needed to activate it.
The sensory cell body is located at the dorsal root ganglion, which is at the level of the neural foramen and distal to the sensory nerve root. The sensory response thus is obtainable with stimulation distal to the ganglion in any lesion proximal to the dorsal root ganglion (preganglionic lesion) despite the loss of sensation in the distribution of the nerve root because of the intact axon distal to the ganglion.
NERVE CONDUCTION INJURIES
Peripheral nerve injuries are most commonly classified according to Seddon’s scheme, which identifies three classes of injury. The terms corresponding to these classes—neurapraxia (temporary loss of nerve conduction without structural change of the axon), axonotmesis (disruption of the axons), and neurotmesis (complete disruption of the axons and surrounding connective tissue)—are generally used by electromyographers (Table 16–1). Sunderland’s classification of nerve injury, although more extensive, is not helpful to the electromyographer and may be more useful to the peripheral nerve surgeon.
Neurapraxia | Axonotmesis | Mixed Injury (Neurapraxia and Axonotmesis) | Neurotmesis | |
---|---|---|---|---|
Motor | ||||
Proximal | Decreased amplitude | Decreased amplitude | Partial decreased amplitude | No response |
Distal | Normal amplitude | Decreased amplitude | Partial decreased amplitude | No response |
Sensory | ||||
Proximal | Decreased amplitude | Decreased amplitude | Partial decreased amplitude | No response |
Distal | Normal amplitude | Decreased amplitude | Partial decreased amplitude | No response |
Demyelination | Present | Absent | Partially present | Absent |
Wallerian degeneration | Absent | Present | Partially present | Present |
Perineurium intact | Yes | Yes | Yes | No |
Amplitude is the most important measure in the nerve conduction study because it reflects the number of conducting nerve fibers. If some of these fibers are blocked (as occurs in neurapraxia), then the amplitude with stimulation proximal to the block will be lower in a manner proportionate to the number of fibers blocked. Latency reflects information about the fastest conducting fibers. Conduction velocity reflects the fastest time between two stimulating electrodes. This measure is not sensitive enough to assess focal axonal loss but measures pathologic changes involving the myelin. Conduction velocities can be normal in patients with focal axon loss neuropathies in which up to 75% of the motor nerve fibers have been lost, especially if the loss primarily affects the slower conducting axons. With proximal stimulation and greater distance from the recording electrode in a normal nerve, there is desynchronization and phase cancellation of the response, which decreases the amplitude of the motor response. Although some physiologic dispersion and loss of amplitude is normal, the loss of amplitude proximally of 25–30% or greater may be evidence of a conduction block in most of the nerves.
The mildest injury, neurapraxia, has the best prognosis for recovery. Neurotmesis, which results in absent motor amplitude throughout the course of the nerve, has the worst prognosis, given the disruption of axons and epineurium that occurs. This problem may require surgical repair by a skilled peripheral nerve surgeon. Outcomes are often guarded, although the surgical approximation of nerve fibers is improving. The prognosis for recovery depends on many factors, including proximity of the lesion to the muscle(s) being innervated, integrity of the muscle itself, and age and health of the patient. In partial axon loss lesions, the previously mentioned factors should be taken into consideration for recovery along with severity of the lesion. In general, upper brachial plexopathy has a better prognosis than lower brachial plexopathy, because of the length of axonal regeneration needed in each instance.
After a nerve injury, changes in amplitude of the compound muscle action potential occur over a period of 6–8 days, and in the sensory nerve action potential over a period of 8–12 days; therefore, nerve conduction studies performed within 1–2 weeks after a traumatic injury can determine whether there is axon loss and, if present, the severity of it. Changes seen on needle electromyography may evolve over 3–4 weeks and are more helpful in localizing the axonal loss lesion (Table 16–2). Electrodiagnostic studies can establish where a lesion is located and whether it is generalized, multifocal, or focal. Additionally, they can indicate whether the underlying process involves demyelination or axon loss.
NCS Changes | ||
Segmental Demyelination | Partial Axon Loss | |
CMAP amplitude | ||
Distal | Normal | Decreased |
Proximal | May decrease (temporal dispersion) | Decreased |
CMAP duration | ||
Distal | Normal | Normal |
Proximal | Prolonged (temporal dispersion) | Normal |
Distal latency | Prolonged | Normal range |
Conduction velocity | Slow | Normal range |
EMG Changes | ||
Segmental Demyelination | Partial Axon Loss | |
Fibrillations and PSW | No | Yes |
MUAP changes | ||
Acute | None | None |
Subacute | None | Normal amplitude/wide duration polyphasic units |
Chronic | None | Large amplitude/wide duration polyphasic units |
MUAP recruitment | Normal | Decreased |
Electrodiagnostic studies are biased toward evaluating the large, myelinated (type A) peripheral nerve fibers, and not the smaller type C pain fibers. Two different types of studies are performed: (1) motor and sensory nerve conduction studies (NCS), and (2) needle electromyography (EMG). The EMG study analyzes motor units and spontaneous potentials seen in the muscles after axon loss nerve injury. NCS and needle EMG together are commonly referred to as “EMG.” These two studies are used in combination to assess and diagnose multiple neuromuscular problems.
The changes noted on NCS or needle EMG are dependent on the type of injury and the time from injury. These tests can help confirm a suspected peripheral nerve injury or, alternately, suggest other explanations for a patient’s pain, muscle atrophy, or weakness. One advantage of early electrodiagnostic testing is its ability to detect evidence of conduction block and continuity of nerve fibers. It can also help to rule out premorbid problems such as underlying peripheral neuropathy, which may influence a patient’s recovery. One disadvantage of early electrodiagnostic testing is its limited prognostic value, as testing may take place before neurophysiologic changes have had time to evolve.
In the hands of a skilled electromyographer, electrodiagnostic studies have a specificity greater than 90%. The results of these studies should always be interpreted in combination with the clinical history and physical examination. An electrodiagnostic study is not an extension of the physical examination; it only confirms a clinical impression. If there are electrodiagnostic findings in the absence of clinical findings, the test does not add to the diagnosis or treatment. When inconsistencies are noted, the clinician should consider whether affected nerves were compared with similar nerves in the contralateral limb, whether the patient has a different underlying medical condition, or whether the electrodiagnostic study was thorough and technically competent. Patients should ideally be evaluated by an electromyographer who can perform a complete electrodiagnostic consultation, interpreting electrodiagnostic results in the context of the patient’s history, physical examination, and other pertinent studies such as imaging.
NERVE CONDUCTION STUDIES
NCS commonly assess either sensory or motor nerves, although mixed nerves can also be assessed using the same methods. These impulses are recorded as sensory nerve action potentials (SNAPs), compound muscle action potentials (CMAPs), or compound nerve action potentials (CNAPs). Measurements of conduction velocity and distal latency are reflective of the speed of propagation of action potentials via large myelinated axons. As such, slowing of these measurements may suggest dysmyelination. The conduction velocity can also be slow in remyelination after dysmyelination, reinnervation after axon loss injury, cold temperature, wallerian degeneration of the fastest conducting fibers, and axon stenosis. Amplitude of SNAPs is reflective of the number of axons in a sensory nerve. CMAP amplitude is reflective of the combined function of the motor neurons, the neuromuscular junction, and the striated muscle.
In NCS, nerves are stimulated percutaneously using electrical impulses. A recording electrode (identified as G1) is placed over the nerve or muscle, a reference electrode (G2) is placed nearby to filter out extraneous electrical activity, and a ground is placed between stimulus and recording electrode to reduce stimulus artifact.
Supramaximal stimulation is an important concept in NCS. A submaximal stimulus will trigger a response in some, but not all, axons in a nerve. A maximal response will activate the entire group of axons. Once this point is reached, further increase in the intensity of stimulation will not change the response. When conducting a study, the electromyographer must increase the stimulus until no change in amplitude is noted between a response at a lower intensity of stimulus and the next higher level. This helps assure that accurate data are being obtained and recorded. Further increase in the stimulus beyond this point decreases the latency owing to distal migration of the cathode.
SNAPs are recorded using two electrodes and a common ground.(Figure 16–3) The active (G1) electrode is placed superficial to the nerve. A second electrode, the reference electrode (G2), is placed 4–5 cm distally. An electrical stimulus is delivered, creating an electrical field that propagates down the length of the nerve. The initial portion of the electrical field is considered positive. As it approaches the G1 electrode, this is recorded as an initial positive or downward deflection in the action potential waveform. As the impulse continues to pass beneath the G1 electrode, a large negative deflection is produced in the waveform. As the impulse travels away from the G1 electrode, a final positive portion of the nerve action potential waveform is seen. The distance between the G1 and G2 electrodes is important and should be at least 4 cm. If the electrodes are too close together, the signal may be distorted, with the beginning of the signal approaching the G2 before the end of the signal passes over the G1; this will cause attenuation of the amplitude.
Figure 16–3
Sensory nerve action potential.
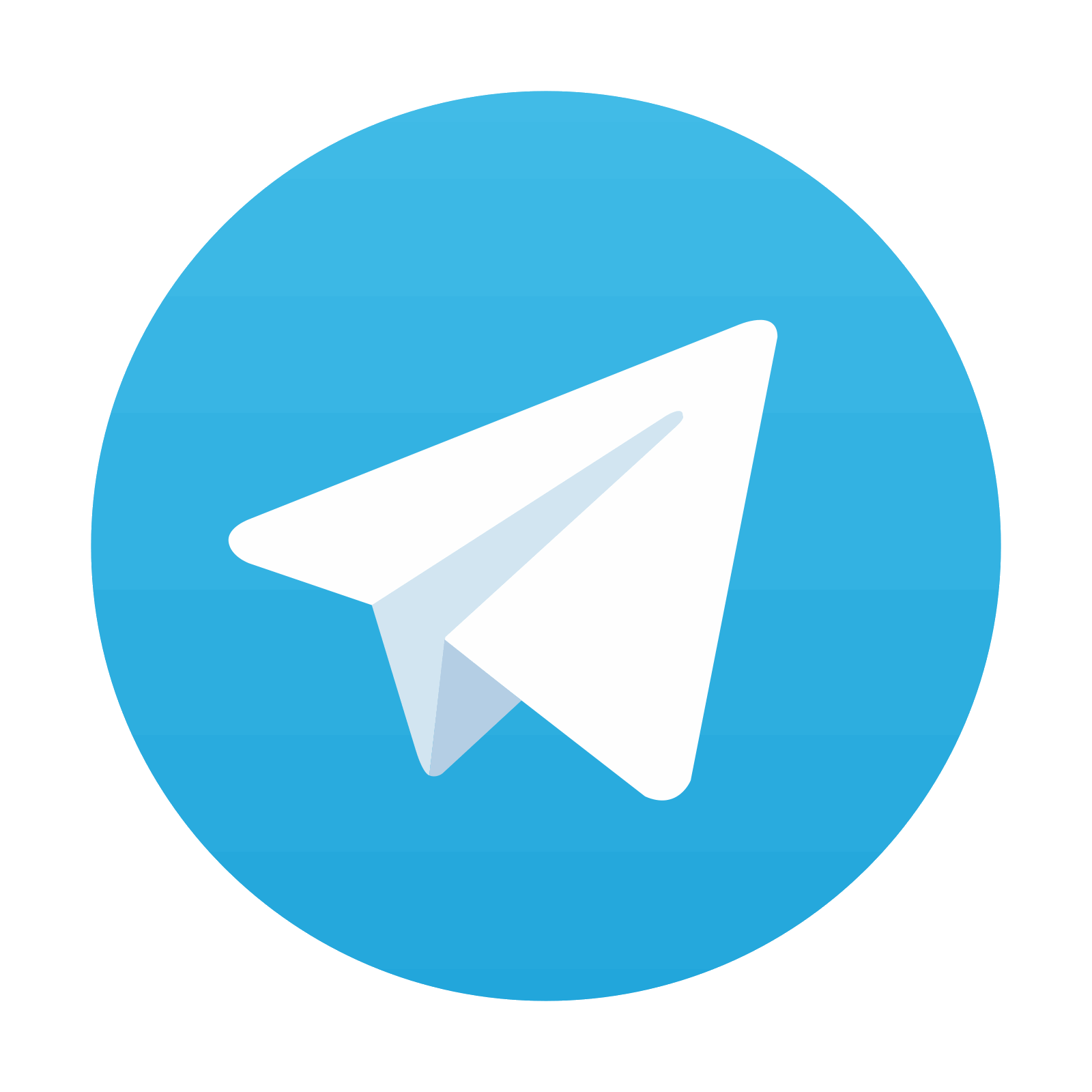
Stay updated, free articles. Join our Telegram channel
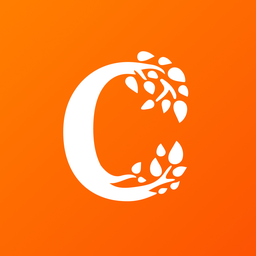
Full access? Get Clinical Tree
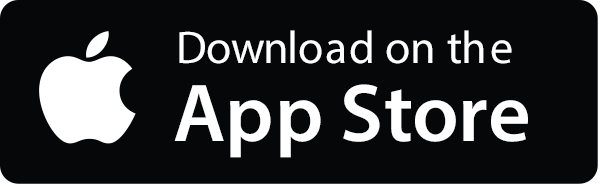
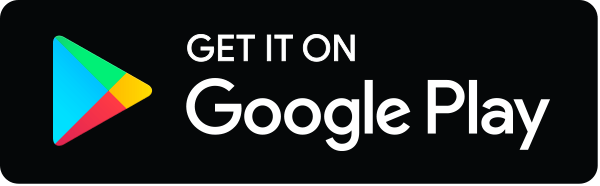
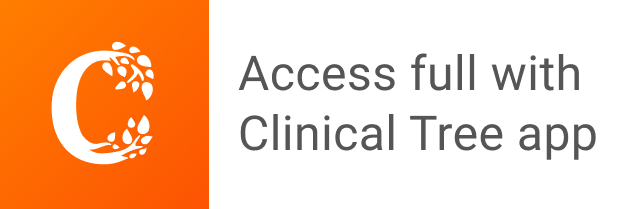