1.3 Implants and biotechnology
To access the videos, please follow the URL link
1 General requirements
The use of metal in fracture fixation has demonstrated unrivaled success for decades owing to the metal′s high stiffness, strength, good ductility, biological toleration (biocompatibility), lack of toxic and inflammatory reactions, and reliable function.
An implant material, which corresponds to international standards, generally manifests an adequate level of biocompatibility (the ability of the material to perform with an appropriate host response in a specific application in a specific location). It should be noted that metals that may behave extremely well mechanically and biologically for fracture fixation in one anatomical area may cause biological problems in another.
The metals currently used are electropolished stainless steel (EPSS) (ISO 5832-1) and commercially pure titanium (cpTi) (ISO 5832-2) [1–3], along with titanium alloys such as titanium aluminum niobium (TAN) (ISO 5832-11). Despite the many differences between these metals, they provide a fairly predictable clinical outcome, and offer comparable success for achieving the main biomechanical and biological requirements of fracture fixation despite clear differences in implant properties and biological responses [1]. Ceramics, polymers, carbon composites, and degradable materials are also used for special applications [3] but not in situations where high loads are to be expected, unless metal fixation is also present.
Implant materials used for internal fixation must conform to certain basic requirements; reliable function and minimal adverse effects for the patient are equally important. The selection of material and implant design must respond to several conflicting requirements. This chapter introduces the basic principles used when selecting materials for internal fixation devices.
2 Material mechanical properties
2.1 Stiffness
Stiffness is defined as the ability of a material to resist deformation. It is measured as the association between the load applied to the material and the resulting elastic deformation of the material.
The stiffness of a material is its modulus of elasticity. The stiffness of an implant results from the modulus of elasticity of the material and the shape and dimensions of the implant itself. As an example, the modulus of elasticity of cpTi (titanium) (110 GPa) is much less than that of EPSS (stainless steel) (186 GPa), and so, under similar load conditions, it will deform more. However, the dimension of the actual implant is also important: increasing the thickness of a standard cpTi plate by a few tenths of a millimeter will increase its bending stiffness. EPSS is a much stiffer material than titanium, which may be relevant for problems relating to normal loading of the adjacent bone. The increased flexibility of cpTi and its alloys means that the elastic deformations are closer to bone (20 GPa) compared with EPSS. Due to this, cpTi and its alloys have increased fatigue resistance compared with EPSS and show superiority over steel under cyclic load [3].
Osteosynthesis restores bone stiffness temporarily until fracture healing restores it permanently.
When we consider an implant (screw, nail, plate, or external fixator) spanning a fracture, the stiffness of the implant must prevent deformity at the fracture site. To allow proper healing the device must reduce fracture mobility to below the critical level at which healing tissue will form. During the biological differentiation cascade, the tissue within the fracture site differentiates from hematoma to granulation tissue to cartilage to bone, with a gradual increase in strength associated with a decrease in the tolerance of strain. Granulation tissue tolerates 100% strain, cartilage 15% and forms under conditions of higher dynamic deformation (strain) than the final mineralized tissue. Cortical bone tolerates 2% strain. Nature gradually increases rigidity and strength and each new tissue forms under the protection of its predecessor (see chapter 1.2).
In the past, attempts have been made to produce implants that have a material stiffness similar to bone, using plastic or carbon reinforced composites [4]. These implants were thought to reduce the stress shielding that was believed to occur with stiff metal implants that take the load away from the bone. Preclinical research combining intravital staining of the vascular system and polychrome fluorescent labeling of bone remodeling demonstrated that early bone porosis in the locality of the implants is the result of internal cortical bone remodeling induced by necrosis rather than by unloading [5]. Perren′s necrosis theory is strengthened by (1) bone porosis is temporary in internal bone remodeling; (2) the pattern of the remodeling zone is closely related to that of the disturbed circulation, and not to that of unloading; (3) plastic plates may produce more bone porosis than steel plates; (4) improved blood circulation using modified plates results in reduced porosis. Implants with low material stiffness do not as a rule offer an acceptable balance between biology and mechanical properties
2.2 Strength
Strength is the ability of a material to resist the application of forces without deformation or failure.
Thus, strength determines the amount of load an implant can resist. Before a metal breaks it may irreversibly deform (plastic deformation). The dimensions of the implant are often more important than the strength of the material. The strength of cpTi is about 10% less than that of steel ( Table 1.3-1 ), but an increase of the implant cross-section can compensate for the difference in material strength. Strength determines the limit of stress (force per unit area), which results in deformation.
For internal fixation, the resistance of an implant to repeated load, which may result in failure by fatigue, is a critical issue.
Compared with steel, cpTi is somewhat less resistant to single loads but greatly superior when high-cycle repeated loads are applied [5].
2.3 Ductility
The ductility of a material is the degree of permanent deformation it tolerates before it breaks (plastic deformation without material fracture).
The ductility of a material determines the degree to which an implant, such as a plate, can be contoured by the surgeon for different anatomical requirements. As a rule, materials of high strength, such as titanium alloys and highly cold-worked cpTi, offer less ductility than steel (though the most common EPSS fracture fixation devices, such as bone screws, plates and nails are cold-worked, which provides an intermediate condition of tensile properties and ductility). Ductility provides some forewarning of impending failure, for instance during insertion of a screw. According to international standards, a 4.5 mm cortex screw (ISO 6475) must tolerate more than 180° of elastic and plastic angular deformation before breakage. However, cpTi, having lower ductility, provides less tactile feedback (pre-warning), which means that the surgeon should first acquire some hands-on experience of the different handling techniques before surgical use.
This limitation of lower ductility of cpTi is now partly overcome by producing anatomically designed implants that do not require contouring (and theoretically should not be contoured) by the surgeon. Contouring can lead to deformation of the thread-bearing part of the combination hole with locking compression plate (LCP) implants, rendering them incapable of retaining locked screws and is therefore not advised.
cpTi and its alloys have two main mechanical conditions, annealed (softer) and cold-worked. The annealed version is used for lower-stress loading applications, such as mini-fragment plates. The cold-worked cpTi (and alloys) has increased strength and is used for plates, screws, and nails.
2.4 Torsional properties
Torsion is the twisting of an object due to an applied torque (the force to rotate an object about an axis, the turning force). One observed clinical benefit of EPSS relates to its torsional properties. When the maximum torque of an EPSS screw insertion is reached, the screw will no longer hold torque properly, bottoms out and no longer advances. Even with continuing screw rotation a constant torque is sustained. The screw head will continue to rotate approximately 1.5 turns until it breaks. For surgeons, this property is an advantage, providing tactile feedback during insertion and limiting the possibility of screw stripping. cpTi (and its alloys) screws do not provide as much of a tactile response. Once the screw bottoms out, it fails to reach maximum torque and continuing rotation of the screw head persists in increasing the torque. Breakage of the screw occurs after approximately an additional three to four turns. Torque-limiting screwdrivers are available to help prevent such problems. A clinically relevant comparison of the torsional performance of EPSS and titanium alloy tibial intramedullary nails was performed using distal locking screw holes and dedicated cross screws to secure each nail distally [6]. From the mechanical testing, the mean torsional rigidity of the titanium alloy nail system was 40.9 N/m2 while that of the EPSS nail system was 34.6 N/m2. Theoretical calculations of the torsional rigidity of the central part of the nail were 83 N/m2 for the EPSS nail and 66 N/m2 for the titanium alloy nail.
This study shows that there are clinically relevant biomechanical properties of the whole implant system. It is not just the material that should be considered.
3 Material biocompatibilty properties
3.1 Corrosion resistance and toxicity
Corrosion is an electrochemical process that results in the destruction of metal by the liberation of ionic metal.
Corrosion differs in implants made of one single component and implant systems with several metal components. Stainless steel, cpTi, and TAN, if tested as a single element (ie, only as a plate or screw and not as a combination of the two), are highly resistant to corrosion, even in the environment of body fluids. This is due to a protective, passive layer which forms on their surfaces. Titanium and titanium alloys have extreme chemical inertness. An oxide passivation film forms on titanium and its alloys that is much more corrosion resistant and thermodynamically stable than the chromium oxide film that forms on stainless steel. The passive layer on titanium is formed quickly, is electrically isolating, and the implant shows literally no corrosion ( Fig 1.3-1 ). Stainless steel is susceptible to crevice corrosion. Cobalt chromium molybdenum (CCM) is more corrosion resistant but can release “toxic” ionic species when depassivated (US Food and Drug Administration [FDA] concern issued February 2012).

Recent in vitro and in vivo research has produced a persuasive case characterizing many constituents of stainless steel implants, such as chromium (Cr), cobalt (Co), iron (Fe), and nickel (Ni), as the main causes of toxicity, the undesirable effects of which extend to a variety of systems such as the vascular, immune, excretory, reproductive, integumentary and nervous systems [1, 7, 8]. The use of titanium and titanium-alloy implants, which have superior function under mechanical/cyclic load compared with EPSS, is an alternative without these concerns. The accelerated regeneration rate of titanium oxide surfaces would afford better resistance to substantial metal ion release compared with EPSS.
Cobalt alloys, which are successfully used in single element endoprosthetic implants, are not currently used in internal fixation implants due to their susceptibility to galvanic corrosion when in contact with steel. Since the polarization plots for cpTi and TAN are similar, the materials could be used for multicomponent device applications; galvanic corrosion would not be expected to occur. On top of the intrinsic corrosion problems, mixing dissimilar materials will likely add the problem of galvanic coupling, though preclinical studies with different metals used for cannulated screws and guide wires could not find adverse in vivo effects [9].
Erosion is a physical process that results in structural degradation of the implant surface, with the release of material debris that ranges in size down to a few nanometers.
In orthopedics, the major form of erosion is fretting corrosion encountered in modular implant systems, such as when a screw head moves in relation to the plate hole. Fretting occurs with micromotion between two adjacent implant surfaces. This results in the release of submicron sized particles into nearby tissue. Fretting particles cause a number of clinical complications. Experimentally produced debris from steel, cpTi, and an alloy Ti-15Mo, when examined in vitro, showed phagocytosis of particles of all three materials by macrophages in a dose-dependent response. Steel particles also inhibited cell proliferation, even when the particles were not in direct contact with the cells and caused cell membrane damage. When two implants made of cpTi are moved against each other under load, metal debris from abrasion may be observed in the local surrounding area (with particle sizes often larger than 10 µm [10]), giving a harmless discoloration in the tissues. Steel wear debris has been observed in organs remote from the site of the implant, showing that it can disseminate around the body [7]. When steel fretting occurs, the particles produced are less than 0.5 µm in size [10] and can be easily transported away from the implant site. If submicron titanium particles are transported away from the implant site, they should not elicit a tissue reaction due to their high biocompatibility. Though cpTi ions have been observed in increased amounts in hair of patients in a study [11] where the patients received spinal implants. To our knowledge, no publications have shown tissue reactions in organs remote from the site of a cpTi implant. For flexible internal fixation where motion and thus fretting is to be expected, titanium or its alloys are the materials of choice.
3.2 Surface properties
EPSS, cpTi, and its alloys differ in their surface oxide composition, which is a major determining factor in protein adsorption, cell attachment, and finally either fibrous-osseous integration or direct osseointegration [1].
When placing an implant into the body, the implant becomes conditioned immediately by water, blood, and protein, minimizing the extent of which the actual implant contacts adjacent tissue. This is followed by either fibrous-osseous integration or direct osseointegration. Without cell adhesion, under the presence of micromotion, fibrous capsule formation occurs [12]. The properties of the implant surface, such as microtopography and chemistry help to define the eventual tissue response via the protein and cell interactions. The influence of surface microtopography continues from the moment of device implantation for several months until a final tissue response has been determined. The reader is referred to a review on this area for further information, including the theory of the “effective roughness spectrum”, a hypothesis for control of cell surface integration based upon the notion that for a surface-mediated cell response to occur it is necessary for the individual cells to perceive the micro-roughness [13]. The development of a stable bone-implant interface is considered critical for the success of osteosynthesis implants, such as screws. The surface structure of an implant in contact (direct osseointegration) or near contact (fibrous-osseous integration) with bone is important because force transmission occurs at this interface. An in vivo study in rabbits showed that simple surface roughness modification of steel internal fixation plates within the “effective roughness spectrum” induced more bone formation toward the implant surface without fibrous tissue formation in between ( Fig 1.3-2 ).

Several studies [14–16] have detected a continuous one- to two-cell thick fibrous cell layer separating EPSS devices from bone (where the EPSS surface topography is outside/below the “effective roughness spectrum”). cpTi (non-polished) and its alloy TAN support direct osseointegration (where the surface topography of cpTi and its alloy TAN are within the “effective roughness spectrum”), without a continuous fibrous interface. A proteoglycan layer has been reported [17], which contains closely packed ordered collagen bundles adjacent to (non-polished) cpTi implants, yet for EPSS this layer does not contain ordered collagen filaments (where surface topography of EPSS is outside/below the “effective roughness spectrum”). This is believed to accelerate osseo-integration to cpTi and its alloys due to the enhanced degradation of the hyaluronan network, formed in wound healing [16]. The 3-D surface micro-morphology with microdiscontinuities of (non-polished) cpTi and its alloys (where the surface topography is within the “effective roughness spectrum”) compared with the smooth surface of EPSS (outside the “effective roughness spectrum”) is thought to offer increased anchorage to the fibrin matrix upon implantation [13]. The distinct fibrous interface formed with EPSS implants does not appear to cause any compromise in implant stability in preclinical studies with bone screws [14], LCPs and locked screws [16], or intramedullary nails [15]. The fibrous interface formed with EPSS implants and polished cpTi and polished titanium alloy implants was observed to generate significantly lower screw removal torque and nail pull-out forces compared with standard microrough cpTi and titanium alloy counterparts [14–16]. Analysis with in vitro research with osteoblasts suggests that the effect of polishing is not only purely mechanical but also has effect at the cellular level [18]. Given the proven success of EPSS in fracture fixation largely without major incident, these numerous research results strongly challenge the unproven myth that direct osseointegration is required for implant stability; fibrous-osseous integration is thought to be sufficient. Modern fracture fixation devices (locking plates and locked nails) attain immediate stability because of the implant design and therefore direct osseointegration is also not essential on such implants for stability. In such cases, surfaces which do not encourage direct osseointegration suffice. Such surfaces will have advantages for implant removal, allowance of free-gliding tissues to glide (eg, tendons, muscles, nerves) and the discouragement of protein, cell and bacteria attachment and reduce implant biofilm formation on osteosynthesis implants.
While (non-polished) cpTi and its alloys would appear to have a clear advantage over EPSS for supporting direct osseointegration, this advantage is compromised in numerous anatomical locations. For instance, the formation of restrictive adhesions between an implant surface and tendons is a large clinical problem in hand surgery [19]. Occurrences of these adhesions appear more common with (non-polished) cpTi implants than with the smooth EPSS implants. The effect of distal radius (non-polished) cpTi implants on the functioning of extensor tendons in a preclinical study [20] demonstrated 100% free gliding of tendons over EPSS implants but only 43% over (non-polished) cpTi. It has been shown that the greater occurrence of liquid-filled capsule formation adjacent to EPSS and polished cpTi implants is due to the smooth surfaces devoid of microdiscontinuities [21]. This effect of surface microtopography has been confirmed in vitro, where increased fibroblast growth and spreading is observed on “smoothened/polished” implants compared with a standard microrough (non-polished) clinical titanium alloy [20]. Clinically, this high attraction of bone for the 3-D microrough surface of cpTi and its alloys can cause an assortment of complications upon removal of the implant, such as screw stripping screw/plate breakage, bone in-growth into the screw-plate interface for locked screws causing great difficulty in removing implants and problems separating the implant from the bone which may result in fracture. Bony integration is minimized by using surfaces with minimal microstructure reducing the forces required to remove screws.
In clinical practice, fibrous capsule formation has been observed to be more prevalent with standard EPSS than with standard (non-polished) cpTi plates ( Fig 1.3-3 ). This is the reason why EPSS allows free-gliding tissues, such as tendons to move easily over the implant surface without clinical problems.

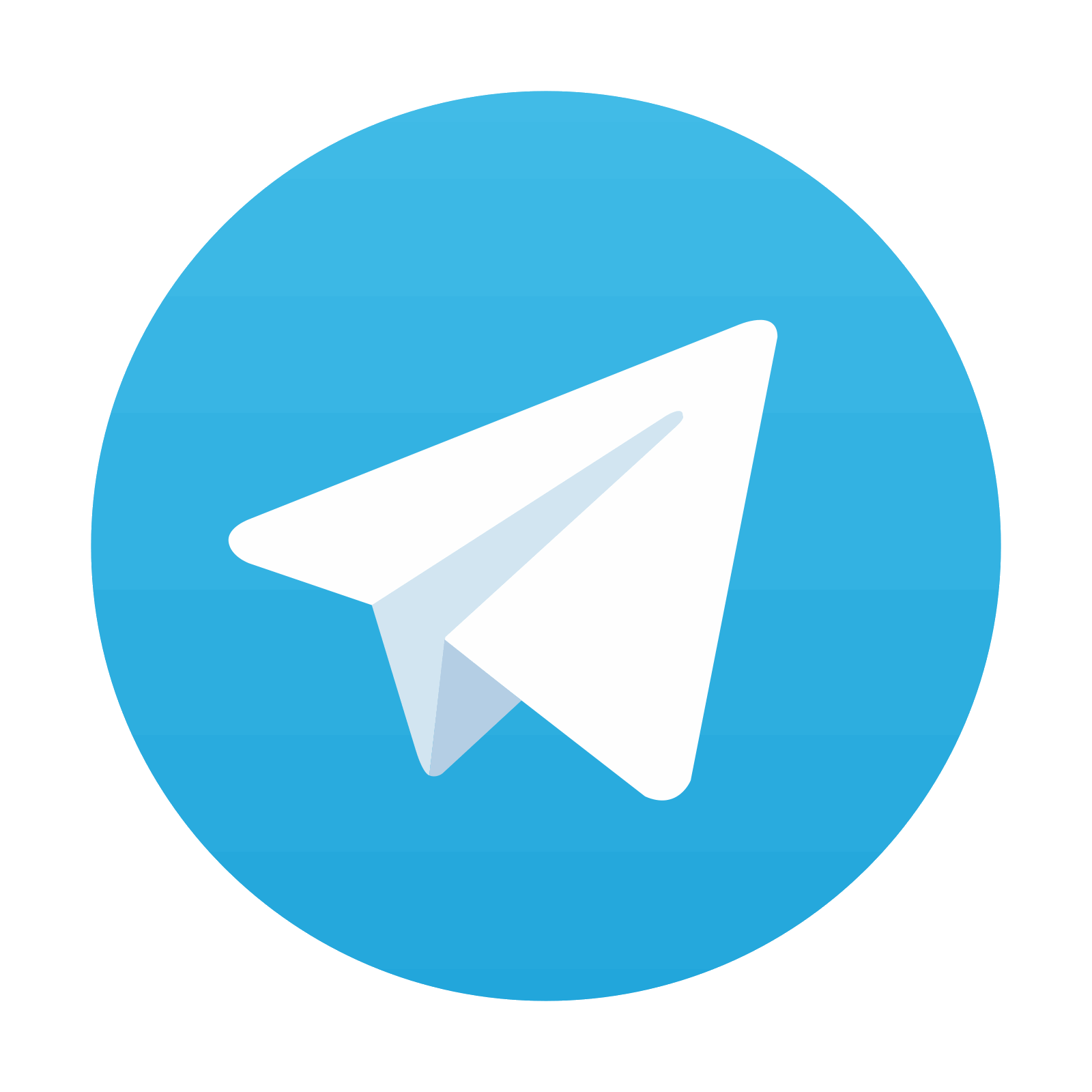
Stay updated, free articles. Join our Telegram channel
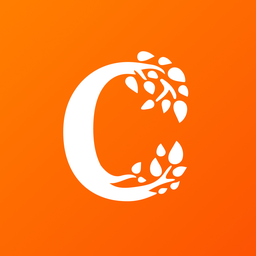
Full access? Get Clinical Tree
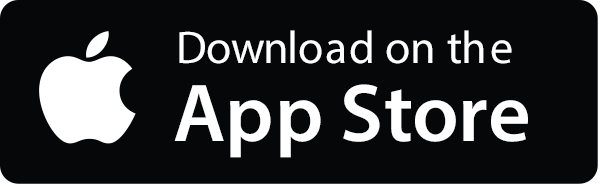
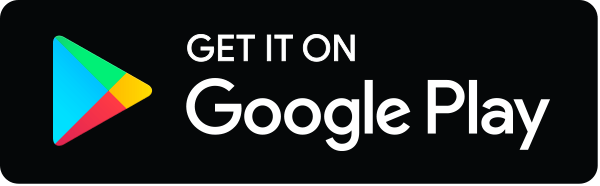
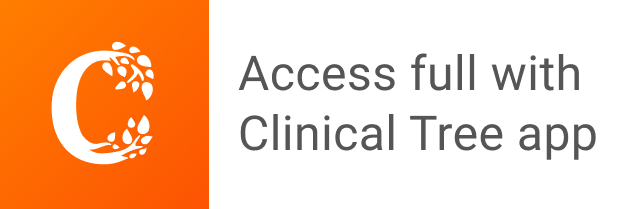